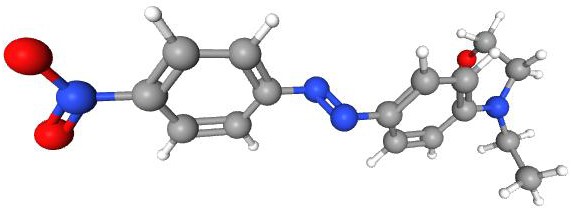
Citation: | Namunakhon NABIYEVA, Tohir AKRAMOV, Davronjon ABDUVOKHIDOV, Yuantao ZHANG, Maksudbek YUSUPOV, Jamoliddin RAZZOKOV. Preliminary study on degradation mechanisms of plasma-treated DR1 by atomistic simulations[J]. Plasma Science and Technology, 2025, 27(3): 035504. DOI: 10.1088/2058-6272/ada21e |
Cold atmospheric plasma (CAP) has emerged as a promising technology for the degradation of organic dyes, but the underlying mechanisms at the molecular level remain poorly understood. Using density-functional tight-binding (DFTB)-based quantum chemical molecular dynamics at 300 K, we have performed numerical simulations to investigate the degradation mechanism of Disperse Red 1 (DR) interacting with CAP-generated oxygen radicals. One hundred direct-dynamics trajectories were calculated for up to 100 ps simulation time, after which hydrogen-abstraction, benzene ring-opening/expanding, formaldehyde formation and modification in the chromophoric azo group which can lead to color-losing were observed. The latter was obtained with yields of around 6% at the given temperature. These findings not only enhance our understanding of CAP treatment processes but also have implications for the development of optimized purification systems for sustainable wastewater treatment. This study underscores the utility of DFTB simulations in unraveling complex chemical processes and guiding the design of advanced treatment strategies in the context of CAP technology.
The growing demand for dyes in industries such as pharmaceuticals, food, and textiles inevitably led to an increase in dye effluents discharged into wastewater [1]. These outflows pose a significant threat to aquatic ecosystems, including plants, animals, and indirectly, human beeings through the consumption of food grown in water contaminated with dyes [2–4]. Due to the intricate organic structures of dyes, conventional treatment methods such as physical, chemical, and biological approaches (including ultrasound, cavitation, UV irradiation, chlorine and ozone oxidation, and microbial treatments) are often ineffective, economically unfeasible, or may generate secondary toxic byproducts [5–8]. Consequently, researchers are exploring alternative methods to address these challenges [9–11].
One such promising approach is advanced oxidation processes (AOPs), which have attracted considerable attention worldwide [12–15]. AOP involves the generation of hydroxyl radicals (HO·) through various mechanisms. These radicals effectively break down dissolved organic compounds in wastewater, converting them into non-toxic substances. In addition to HO·, other oxidizing species such as ozone (O3) and hydrogen peroxide (H2O2) also play a vital role in AOPs [16–19]. Cold atmospheric plasma (CAP) technology, a subset of AOPs, stands out among its counterparts due to its eco-friendliness, ease of production, and economic viability [20–24]. Unlike other AOPs, CAP does not necessitate costly catalysts, chemicals, or radiation [25–27]. CAP technology uses the power of ionized gas to generate reactive species capable of breaking down organic contaminants in wastewater. Unlike traditional plasma technologies, CAP operates at near-ambient temperatures, making it suitable for treating heat-sensitive materials without causing thermal damage. Additionally, CAP treatment does not require the use of chemicals or produce harmful byproducts, making it an eco-friendly option for wastewater treatment [28, 29].
Although CAP devices consume electrical power, their environmental footprint/impact is significantly reduced compared to other AOPs. The resources required for CAP primarily include the manufacturing cost of the plasma device, power supplies, and electrical energy as operational expenses.
The degradation of organic dyes using CAP has been the subject of considerable research interest with studies mainly focusing on different types of CAP devices, such as dielectric barrier discharge (DBD), plasma jet, gliding arc and corona discharge [30–33]. Rahimpour et al investigated the removal of crystal violet dye from a liquid using a post-discharge pulsed DBD reactor, with O2 as the working gas [34]. In their study, oxygen was introduced into the DBD reactor, and the resulting effluent gas was bubbled through the solution. This approach enhanced the mass transfer of reactive oxygen species (ROS) into the solution, leading to a higher degradation rate of the dye. When argon was added to the working gas at a fixed input power, the dye degradation increased compared to using pure oxygen. This improvement was attributed to the formation of more ozone molecules due to the higher dissociation of oxygen molecules facilitated by argon metastables and energetic electrons in the plasma system. The degradation kinetics of crystal violet followed a first-order reaction (i.e., a type of chemical reaction where the rate at which a dye is decomposed is directly proportional to the concentration of the dye). Complete elimination of total organic carbon was achieved after the plasma treatment, indicating full mineralization (i.e., degradation into simpler inorganic substances) of the treated solution and the removal of unwanted organic carbon. Abdelmalek et al studied the decomposition of Orange I, Crystal Violet, and Eriochrome Black T dyes in aqueous solutions using a gliding arc discharge [35]. The plasma was generated between two diverging electrodes with the introduction of humid air. They found that the degradation of all three dyes followed first-order kinetics (i.e. the rate at which the dye concentration decreases over time is proportional to its current concentration). Additionally, they reported that the mineralization of the dyes decreased as the plasma exposure time decreased. In the study by Sun et al [36], a multi-needle jet with 24 needle electrodes was used to produce pulsed plasma for treating an aqueous solution containing methyl orange dye with different working gases (oxygen, air, argon, and nitrogen). A plate disc placed inside the dye solution served as the grounded electrode, and the plasma was formed in the gaseous phase between the needle electrodes and the dye solution. The decoloration rate followed the order: oxygen > air > argon > nitrogen. Additionally, it was reported that a smaller discharge gap was more effective for degradation due to the enhancement of the electric field in the gap, which leads to the formation of a large number of reactive species. Magureanu et al [37] investigated the removal of methylene blue from aqueous solutions using a pulsed high voltage corona discharge with multi-hollow needle electrodes arranged in a six-needle array. These needle electrodes were positioned at the bottom of the reactor, with a grounded electrode placed about 5 cm above their tips. Both electrodes were submerged in the liquid, and the discharge occurred in bubbles within the dye solution. The study examined the results of using different feed gases (air, argon, and oxygen) bubbled through the needles into the solution. The multi-needle electrodes generated a large volume of plasma, enhancing the interaction between reactive species and the dye solution. It was found that, at the same external power input, the degradation of methylene blue followed the order: oxygen > argon > air. The researchers explained that the more efficient degradation in oxygen and argon compared to air was due to the fact that in these gases more energy was used to form reactive species, whereas in air some of the energy was spent on the dissociation of nitrogen molecules. Additionally, they reported that the degradation rate of methylene blue depended on the initial dye concentration and the solution volume, with higher dye concentrations degrading more rapidly in smaller volumes.
In recent years, significant progress has been made in understanding the mechanisms of CAP-induced degradation of organic pollutants in wastewater. Numerous studies have demonstrated the efficacy of CAP technology in treating various types of organic pollutants, including dyes, pharmaceuticals, and persistent organic pollutants [38, 39]. This has led to the development of innovative CAP reactor designs and process optimization strategies aimed at enhancing treatment efficiency and scalability. Kooshki et al highlighted in their study the significance of optimizing the plasma device for selective production of reactive oxygen and nitrogen species (RONS) in water to enhance both operational and economic efficiency in dye wastewater treatment [40]. They introduced a novel method for tuning RONS production. Specifically, using ceramic electrodes (Ce) and operating at higher reactor temperatures led to increased H2O2 production in plasma-activated water, reaching up to 16 mg/L within 30 min, while keeping nitrite production minimal. On the other hand, using copper electrodes with a water-cooling system (Cuw) resulted in higher nitrite concentrations, up to 80 mg/L within the same period, but negligible H2O2 production. The study also found that selective RONS production plays a crucial role in methylene blue dye degradation. The Ce setup demonstrated efficient dye degradation at a rate of 1.04 g/kWh, whereas the Cuw setup was less effective and generated additional by-products in water due to nitration mechanisms. Furthermore, a comprehensive cost estimation analysis revealed that scaling up the plasma technology could operate at lower energy costs compared to AOPs. Selective RONS production for dye degradation could lead to nearly a 30% reduction in total costs.
Central to the success of CAP treatment is the generation and utilization of ROS to initiate degradation reactions. The utilization of atomistic oxygen radicals, in particular, holds significant potential owing to their high reactivity towards organic pollutants [41, 42]. In this context, the computational investigation of specific molecular interactions becomes indispensable, allowing for a deeper understanding of reaction mechanisms [43–45].
Within this framework, density functional based tight binding (DFTB) methodology presents a powerful computational tool for simulating chemical processes with high accuracy and efficiency [46–48]. By employing DFTB, researchers can explore the energetics and dynamics of complex chemical reactions involved in CAP treatment, providing valuable insights into the degradation mechanisms of organic pollutants [49]. Xu et al [50] discussed the complex formation mechanisms of H2O2 resulting from the interaction of O atoms with water when exposed to CAPs. They noted that prior molecular dynamics (MD) simulations using reactive force fields and DFTB methods did not observe H2O2 formation. In their work, they employed density functional theory (DFT) in MD simulations, running 192 trajectories of a system containing 63H2O−O to investigate the reaction mechanisms of O radicals in water. Their calculations revealed that triplet (ground) state oxygen was not reactive. They observed a transient oxywater-like structure, O−OH2, which further evolved into perhydroxyl anion (O−OH−) and its counterpart hydronium ion (H3O+). In most cases simulated, H2O2 was ultimately produced. The formation pathways of H2O2 were found to be highly complex for such a simple hydrogen-bonded system. They classified the mechanisms based on the sources and pathways of the H atom in the H2O2 molecule into: (i) H-abstraction; (ii) H-transfer (n=3,4,5,6,7,8); (iii) proton-delivery (n=2,3); (iv) proton-transfer. Their study confirmed that quantum MD simulations are more accurate for predicting the reaction mechanisms of H2O2 formation in such systems. Dabagh et al [51] conducted a study to evaluate the effectiveness of treated Carpobrotus edulis (TPCE) as a biosorbent for removing the dyes orange G (OG) and crystal violet (CV) from aqueous solutions. They characterized TPCE using FT-IR, specific surface area (Ss), point of zero charge (pHz), and SEM-EDX. The researchers tested the influence of various parameters – such as biosorbent dose, contact time, initial dye concentration, temperature, and pH – using a Taguchi experimental design with an L8 orthogonal array (five parameters at two levels). The DFTB method indicated strong binding of dyes to the adsorbent surface. Monte Carlo and MD simulations also demonstrated significant interactions between the dye and adsorbent surface.
In the study of Kandjani et al molecular dynamics simulations investigate reactions within a low-pressure argon-diluted methane plasma [52]. Initial molar fractions, determined by a 1D fluid model, serve as input for the simulations. Examining reaction pathways at various temperatures (300–1000 K), the study finds that higher temperatures promote the formation of diverse molecules. The progression of species such as C2H and CH3 reveals C2H as a primary precursor and CH3 as a key intermediate in forming larger molecules, clarifying pathways of plasma-induced molecule synthesis.
In this study, we focus on the degradation of Disperse Red 1 (DR1), a commonly encountered organic dye pollutant in wastewater, employing oxygen radicals (or O atoms) as reactive species. DR1 is an azo dye characterized by a structure wherein nitrobenzene is substituted at the 4-position of the phenyl group with a 4-N-ethyl-N-(2-hydroxyethyl)phenylazo group [53, 54]. It serves a dual role as both a dye and an allergen. This compound is classified as a monoazo compound and is a constituent of azobenzenes. The presence of these functional groups makes DR1 highly stable and vividly colored, but also poses challenges for its degradation. DR1 is extensively used in textile industries, poses significant environmental risks due to its persistence and potential toxicity [55, 56]. Through DFTB simulations, we aim to elucidate the intricate molecular-level interactions governing the degradation process of DR1 in the presence of O atoms generated by CAP sources.
The structure of this article is as follows. Section 2 provides information about the simulation setup, detailing the computational methodologies and parameters employed in the DFTB simulations. Subsequently, section 3 presents the simulation results and discussions, wherein we analyze the reaction pathways, energetics, and dynamics of DR1 degradation. Finally, section 4 offers concluding remarks that summarize key findings of our study, highlighting their implications for the development of advanced CAP strategies for wastewater treatment.
To gain deeper insights into the impact of ROS on the plasma-treatment process of DR1 dye and to offer atomistic-level observations of the detailed degradation mechanisms, we conducted reactive MD simulations. For this purpose, we used the DFTB method [46, 47, 57–59]. DFTB is a semi-empirical approach derived from DFT, where one considers electron interactions primarily with nearby atoms, simplifying the Hamiltonian used in calculations. DFTB employs a minimal valence-only electron basis set composed of Slater-type orbitals, which are mathematical functions used to describe the spatial distribution of electrons [57]. This minimal set is optimized for valence electrons, the outermost electrons involved in bonding. The DFT ground state electron density is denoted as ρ, the energy around a reference density ρ0 is expanded in terms of density fluctuations δρ=ρ−ρ0 in the corresponding orders as
E[ρ]=E0[ρ0]+E1[ρ0,δρ]+E2[ρ0,δρ2]+E3[ρ0,δρ3], | (1) |
where ρ0 is chosen to be the superposition of neutral atomic densities. The methods constructed in this way can contain terms up to the first, second or third order and are designated DFTB1, DFTB2 (or SCC-DFTB), DFTB3 respectively. In DFTB, the individual energy contributions are made simpler by the use of parameters. The second-order term E2 includes the Hubbard parameter U, which reflects the chemical hardness of atoms. The third-order term E3 requires derivatives of U. Calculating the zeroth-order term E0 requires a minimal atomic basis set, involving two parameters per atom type.
The zeroth-order term E0 in DFTB is found to be consistent across all three versions of the software, namely DFTB1, DFTB2 and DFTB3. Nevertheless, it is possible to achieve practical improvements in accuracy by optimizing confinement radii, which affect orbital overlaps and Hamiltonian matrix elements and, as a consequence, alter the total energy. The “MIO” parameter set was developed for DFTB2 with these optimized radii. For DFTB3, a reoptimized set, designated “3OB”, was created with the objective of minimizing overbinding and providing a more accurate representation of van der Waals distances [59].
In the DFTB3 framework, Kohn-Sham molecular orbitals (Ψi) are expanded as a linear combination of atomic orbitals ϕμ: Ψi=∑μci,μϕμ. This expansion gives an expression for the total energy which includes terms for the reference energy, repulsive interactions and higher order contributions associated with electron-electron interactions [47]:
EDFTB3=E0+E1+E2+E3=occ∑ini∑μ∈a∑ν∈bciμciνH0μν+12∑a,bVrepab+12∑a,bΔqaΔqb γab+13∑a,bΔq2aΔqbΓab, | (2) |
The Hamiltonian matrix elements H0μν are calculated using the Perdew-Burke-Ernzerhof functional and stored in Slater-Koster files for practical use. The second order interaction kernel γab and its charge derivative Γab=∂γab∂qa arederived to account for electron-electron interactions in thesystem. In addition, the pairwise repulsive potentials Vrepab are empirically fitted and tabulated as splines to facilitate efficient evaluation during simulations [59].
In the context of DFTB3, the LCAO-MO (Linear Combination of Atomic Orbitals - Molecular Orbitals) coefficients ciμ, ciν and the Mulliken partial charges Δqa, Δqb are obtained by solving the secular Kohn-Sham equations:
∑νciν(Hμν−εiSμν)=0 ∀μ,i, | (3) |
where Sμν is the overlap matrix representing the overlap between the atomic orbitals ϕμ and ϕν [58].
The Mulliken charges affect the updated Hamiltonian matrix elements:
Hμν=H0μν+Sμν∑cΔqc(12(γac+γbc)+13(ΔqaΓac+ΔqbΓbc)+Δqc6(Γca+Γcb)), | (4) |
and equation (3) must be solved iteratively until self-consistency is reached.
As reported in reference [49], DFTB3’s computational cost is more expensive than classical reactive MD techniques like ReaxFF-MD; despite its computational complexity, DFTB3 accurately captures hydrogen binding energies and hydrogen transfer barriers without compromising speed and robustness. Additionallay, DFTB3 excels in describing resonance structures, a capability absent in ReaxFF-MD, making it a valuable tool for examining reaction process in molecular levels/scales [60].
In this investigation, we applied the third-order self-consistent charge density functional tight-binding (DFTB3) method [47]. To characterize the interatomic interactions within our DFTB3-MD simulations, we utilized a parameter set known as “3OB-3-1”, specifically designed for DFTB3 and applicable to organic and bio-molecules [61, 62].
As our model system, we utilized the DR1 structure, a molecule consisting of 41 atoms (molecular formula C16H18N4O3, MW: 314.34 g/mol) as illustrated in figure 1.
DR1 is an azo dye characterized by its chromophore group (−N=N−) and two benzene rings (chromogens), along with auxochromes that enhance its solubility and color properties. We considered DR1 as the target object and investigated the interaction between DR1 and atomic O, one of the reactive species produced by CAP. O radicals are favored in CAP applications due to their high reactivity, ease of production, ability to selectively produce desired reactive species, strong oxidative power, energy efficiency, and reduced risk of unwanted by-products. These advantages make them ideal for various applications, including water treatment, disinfection, and pollutant degradation. To comprehensively explore all potential bond-breaking and formation processes resulting from the impact of an O atom, and to generate some statistical insights, we conducted a substantial number of DFTB-MD simulations. Specifically, we performed 100 DFTB-MD simulations to probe the conceivable reaction mechanisms associated with the interaction of an O atom with DR1. Each simulation involved a single O atom impact, allowing us to derive statistical information on the occurring mechanisms of formed byproducts.
Note that we excluded consideration of the aqueous layer enveloping the DR1 molecule due to the high computational cost associated with the DFTB method: the inclusion of the water layer would result in excessively long calculation times [49]. Furthermore, as stated in the introduction, our investigation focused solely on the impact of an individual ROS, i.e., the O atom without consecutive impacts. This approach aimed at elucidating the influence of an individual O atom on oxidation process of pigments and assessing the closeness of simulation results to possible experimental results.
To start the simulations, the DR1 molecule was positioned at the center of a cubic box measuring 30 Å × 30 Å × 30 Å. The process of geometry optimization of the molecule was then conducted using the conjugate gradient algorithm. Subsequently, the system underwent equilibration/thermalization for 103 ps within an NVT ensemble (a system with a constant number of particles N, volume V and temperature T). The Berendsen thermostat with a coupling constant of 102 fs was employed during this process. The equilibration/thermalization process was realized up to the temperature T=300 K when the system reached it. The number of time-steps was 2×106 and the Velocity Verlet method was used over integrations. A time-step of Δt=0.5 fs was utilized in all simulations, including thermalization and subsequent particle impact simulations. Periodic boundary conditions were applied in all three spatial dimensions. A finite Fermi-Dirac electronic temperature Te=1000 K was used within DFTB. This allows for more accurate modeling of radical species and avoids incorrect ionic dissociation, making it a powerful tool for studying complex chemical reactions involving O radicals [63].
Following the equilibration, a single O atom was randomly introduced around the structure, maintaining a minimum distance of 5 Å from the molecule. It is worth to note that the box size was sufficiently large to introduce a single O atom with a random position around the molecular structure. This precautionary step (i.e., positioning the O atom at least 5 Å from the molecule) aimed to prevent initial long-distance interactions between the O atom and the DR1, particularly in terms of Coulomb and van der Waals interactions. The impact simulations constituting 100 DFTB-MD runs were conducted: each one was done for a total simulation time of t=100 ps, equivalent to the number of time-steps 2×105. This duration was deemed sufficient for observing bond breaking and formation events within the DR1 structure. All simulations were carried out using the DFTB+ program package [59, 64].
As indicated in the previous section, we conducted DFTB-MD simulations to explore trajectories of O atoms interacting with the DR1 molecule (see figure 1). These simulations permit to attain a more comprehensive understanding of the oxidation process and the molecular mechanisms underlying this synthetic dye degradation. Figure 2 illustrates the time evolutions of the temperature (a) and total energy (b) of the DR1 system. As shown in the figure, a simulation time of 103 ps was sufficient to achieve a well-thermalized DR1 system. Thereafter, a total of 100 DFTB-MD simulations were performed and as a result, such number of different trajectories were obtained illustrating the impacts between O atom and DR1 molecule. The results are outlined in table 1 including 31 reaction mechanisms. The occurrence frequency of each reaction is given in column 4 of the table indicating how often each process was observed.
Number | Event | Frequency | Details | |
1 | H-abstraction | C8−OH | 3 | Formation of hydroxyl group |
2 | C8−OH | 7 | ||
3 | H-abstraction | C11−OH | 8 | |
4 | C11−OH | 1 | Formation of hydroxyl group | |
5 | C11O1−OH | 3 | Formation of hydroperoxide group | |
6 | C11 and O1H; H2O | 3 | Formation of aldehyde group | |
7 | H-abstraction | C14−OH | 16 | Formation of hydroxyl group, see figure 4 |
8 | H-abstraction | C9−OH | 1 | Formation of hydroxyl group |
9 | C9−OH | 1 | ||
10 | H-abstraction | C12−OH | 5 | |
11 | C13−OH | 3 | Formation of hydroxyl group | |
12 | C15−OH | 2 | ||
13 | C16−OH | 2 | ||
14 | H-abstraction | C19−OH | 1 | |
15 | C22−OH | 3 | Formation of hydroxyl group | |
16 | C23−OH | 1 | ||
17 | H-abstraction | H2O and CH2O | 12 | Formation of water molecule and formaldehyde (see figure 5) |
18 | O-addition | N6−O | 3 | Addition of oxygen to the azo group (see figure 6(a)) → may lead to loss of color |
19 | N5−O | 3 | ||
20 | O-addition | C20−O−C23 | 3 | Addition of oxygen to the benzene ring (see figure 6(b)) |
21 | C16−O−C17 | 1 | Ring-expansion | |
22 | C21−O−C22 | 2 | ||
23 | C10−O−C13 | 1 | ||
24 | C13−O−C16 | 1 | ||
25 | C10−O−C12 | 1 | ||
26 | C18−O−C20 | 3 | ||
27 | O-addition | — | 5 | Benzene ring-opening (see figure 6(c)) |
28 | O-addition | C19−O | 1 | Addition of oxygen to C19, detachment of H connected to C19 and binding of it to N5, formation of ketone group |
29 | O-addition | N7O3−O | 2 | |
30 | C21=O and NO2 | 1 | Formation of C21=O bond, dissociation of C21−N7 bond and subsequent detachment of NO2 from the system | |
31 | O-addition | N4+O | 1 | |
Total number of events | 100 |
As mentioned above, DR1 possesses a chromophoric azo group (−N=N−) as a key contributor to its color, see figure 3. Additionally, the naphthalenol moiety and the surrounding aromatic structures in the molecule contribute to the overall coloration, with the extended conjugated system allowing absorption of visible light and imparting the characteristic red hue to the compound [53, 54].
Numerical calculations reveal that all reactions initiate with H-abstraction from a specific site on DR1, excluding those involving azo groups and ring-breaking of benzene. Put differently, the oxygen atom extracts a hydrogen atom (or in certain instances, two hydrogen atoms) from the molecular structure, resulting in subsequent dissociation or formation.
The reaction mechanism most frequently observed, denoted as reaction (7) in table 1, is visually depicted in figure 4. The oxygen atom extracts a hydrogen atom from the hydroxyl group attached to C14 (indicated by the red arrow in figure 4), resulting in the formation of two radicals: C14· and ·OH Following this, these two radicals combine to form a new hydroxyl group. This category of reaction mechanism, involving H-abstraction followed by the binding of hydroxyl (OH), may also manifest in alternate sites within the DR1 molecule and emerged as the predominant reaction mechanism observed in our simulations (refer to reactions (1)–(17) in table 1). Our simulations did not anticipate the experimentally observed configuration with two or three extra oxygen atoms, as we did not investigate the consecutive impacts of oxygen atoms. However, it is plausible that oxygen addition (i.e., the generation of a new hydroxyl group) could occur subsequently at different locations within the system, potentially leading to the formation of the structures mentioned above.
Another reaction mechanisms most frequently observed, namely reaction (17) as documented in table 1, is visually represented in figure 5. The oxygen atom extracts a hydrogen atom from the alcohol group attached to C11 (indicated by the red arrow in figure 5), leading to the creation of two radicals: C11−O· and ·OH. This initiates a rearrangement process, disconnecting C11−O from the DR1 radical and resulting in the formation of a formaldehyde molecule, CH2O. The ·OH radical then takes the hydrogen bound to C8, eventually leading to the formation of a water molecule in the system. Note that the formed DR1 radical after both the rearrangement and pulling out H bound to C8 is not necessarily stable, that is, it can revert back, but radical molecules also notoriously rearrange, eliminate or fragment in the following steps until more stable radicals are formed. Radical molecules, notorious for their dynamic nature, are prone to undergo further rearrangements, eliminations, or fragmentations in subsequent steps, ultimately converging towards configurations of greater stability. These initial reactions, often regarded as the “first step”, play a pivotal role in elucidating the onset of reaction mechanisms, which remain considerably challenging to explore through experimental observations. This kind of reaction mechanism, encompassing H-abstraction, subsequent binding of OH, and rearrangement reactions, exhibits versatility in occurrence across various positions of the DR1 molecule. Notably, it emerged as second the most frequently observed reaction mechanism cases in our simulations, as evidenced by reaction (17) in table 1.
In figure 6(a), we unveiled a pivotal reaction mechanism wherein the oxygen radical intricately binds to the chromophoric groups inherent in the DR1 molecule, notably targeting the azo group. This catalytic interaction causes a profound structural shift within DR1, which subsequently has a profound influence on its color dynamics. Furthermore, in figure 6(b), the emergence of a benzene ring transformation into a heptagon, i.e. a seven-membered ring structure is visually captured, shedding light on a noteworthy structural transformation within the compound. In particular, the oxygen radical binds to two carbon atoms in the benzene ring, forming a bridge. This subsequently leads to the cleavage of the C−C bond, resulting in the formation of a seven-membered ring. Conversely, figure 6(c) illustrates a new type of phenomenon – a ring opening, hinting at the complex dynamics underlying molecular rearrangements in DR1.
Our predictive analyses extrapolate that such reactions are likely to induce a significant shift in the compound’s coloration, suggesting either a complete disappearance or a noticeable alteration in its hue. This insight underscores the intricate interplay between chemical transformations and the perceptible properties of dyes. By leveraging these visual representations and predictive insights, we endeavor to unravel the complex mechanisms governing the behavior of DR1, thereby deepening our understanding of its chemical intricacies and broadening its potential applications across diverse scientific domains. In addition to the observed reactions, we have identified several other noteworthy reactions. These include instances where oxygen radicals interact with nitrogen atoms at positions N7 and N4 (see reactions (29) and (31) in table 1, respectively), leading to chemical modifications within the molecule. Furthermore, oxygen radicals bind with C21, resulting in the formation of NO2 and subsequent detachment of it from C21. Additionally, another reaction (i.e., reaction (28) in table 1) involves the combination of oxygen radicals with C19, leading to the transfer of a hydrogen atom from C19 to N5. These reactions contribute to the complex array of transformations occurring within the molecular structure.
The degradation of DR1 dye using the CAP mechanisms leverages the reactivity of O· radicals generated during the plasma process. These radicals initiate various reactions that break down the dye’s complex molecular structure. This includes:
● Attack on azo group (−N=N−): O· radicals are highly reactive and target the azo group, most likely cleaving the nitrogen-nitrogen double bond, which was not observed during the simulation time used in our study (i.e., 100 ps). It should be mentioned that in our DFTB-MD simulations, we applied non-consecutive (discontinuous) impacts of oxygen radicals, where each MD run begins with an intact dye molecule. We refer to this approach as simulating a low plasma dosage. As a result, under these conditions, we did not observe any cleavage of the N=N double bond, indicating that the low plasma dosage is insufficient to break the N=N bond responsible for the dye’s color. To determine whether consecutive impacts (where an oxygen atom interacts with a dye molecule already modified in a previous MD run) – representing a high plasma dosage – would affect N=N bond cleavage, we conducted test simulations. These test runs (not shown here) did result in N=N bond cleavage, effectively accelerating molecular degradation. Thus, a high plasma dosage is necessary to cleave the N=N bond, which leads to dye decolorization and eventually results in its degradation.
● Interaction with chromogens (benzene rings): following the cleavage of the azo bond, O· radicals can attack the benzene rings. This leads to the formation of smaller, less complex molecules. The oxidative cleavage of these aromatic rings is essential for complete mineralization of the dye.
Auxochromes in DR1, such as hydroxyl (−OH) and amino (−NH2) groups, influence the dye’s reactivity. They can enhance the dye’s interaction with reactive species, facilitating the breakdown of the molecular structure. The interactions between O· radicals and auxochromes can lead to the formation of intermediate compounds, which further degrade into harmless end products like H2O and CO2.
During the degradation process, various intermediates are formed:
● Perhydroxyl anion (O−OH−): this intermediate is formed during the initial stages of oxidation and can participate in further reactions leading to the formation of HO· radicals.
● Hydronium ion (H3O+): this ion forms alongside perhydroxyl anion and contributes to the acidic environment that can enhance further degradation reactions.
● Hydroxyl radicals (HO·): these radicals are highly reactive and play a significant role in breaking down the remaining molecular fragments, leading to complete mineralization.
Our results can be contextualised with those of reference [56], where da Silva Leite et al evaluated the ecotoxicity and degradation pathways of the azo dye DR1 using the photo-Fenton process. Their analysis, using HPLC-DAD and LC-MS/MS, showed that early stages of degradation increased toxicity due to intermediates, while prolonged treatment eventually reduced toxicity. The study identified most of the intermediates as products of hydroxyl radical addition to the aromatic ring, with some azo bond cleavage. In contrast, our study modelling CAP-induced degradation suggests a different degradation pathway. We observed that the degradation of DR1 under CAP treatment is faster and involves reactive oxygen species. Interestingly, we also identified intermediates that retain the azo group, which is partially consistent with photo-Fenton results. However, the development of toxicity under CAP treatment appears to be different. While da Silva Leite et al emphasised the adjustment of reaction time to control toxicity, our computational results suggest that optimizing the intensity and duration of CAP treatment could similarly minimise toxic by-products. Toxicity monitoring is therefore essential for both treatments to ensure environmentally safe degradation.
This study offers comprehensive insights into the interaction mechanisms of Disperse Red 1 (DR1) with oxygen radicals (a key ROS component) generated by cold atmospheric plasma (CAP), employing reactive DFTB-MD simulations. The research revealed that the primary initiation step involves hydrogen abstraction by oxygen radicals, a mechanism that plays a crucial role in facilitating subsequent dissociation and molecular rearrangements. Notably, the most common reactions involved hydrogen abstraction from various locations on the DR1 molecule, resulting in the formation of new hydroxyl groups and other reactive intermediates. Additionally, a significant secondary mechanism included hydrogen abstraction, leading to the formation of formaldehyde and water, which underscored the complexity of radical-driven rearrangement processes.
Importantly, the study also highlighted key interactions between oxygen radicals and the chromophoric azo group, as well as transformations of benzene rings, resulting in the formation of seven-membered rings or ring openings. These structural changes are predicted to alter or eliminate the color of the compound, reflecting the profound impact of CAP treatment on DR1’s chemical properties.
While the simulations focused on low plasma dosages, which did not show cleavage of the N = N double bond, test simulations indicated that high plasma dosages can break this bond, accelerating decolorization and complete dye degradation. This finding emphasizes the importance of optimizing CAP treatment intensity to achieve effective pollutant breakdown while minimizing toxic by-products.
Our computational results were compared with experimental findings. While both approaches revealed the formation of intermediate products, our study demonstrated that CAP treatment can result in faster degradation with distinct intermediate profiles. This comparison underscores the potential for tailored CAP strategies to efficiently degrade complex dye structures, guiding future research and experimental validation for safe and sustainable wastewater treatment.
Overall, this work advances our understanding of DR1 degradation under CAP, paving the way for further integration of computational and experimental approaches to refine treatment processes, optimize plasma parameters, and address emerging environmental concerns.
Namunakhon NABIYEVA, Tohir AKRAMOV and Davronjon ABDUVOKHIDOV analyzed the data. Tohir AKRAMOV and Davronjon ABDUVOKHIDOV drafted the manuscript, while Yuantao ZHANG, Maksudbek YUSUPOV and Jamoliddin RAZZOKOV provided critical revisions. Maksudbek YUSUPOV contributed to the preparation of the model system and conducted the MD simulations. Jamoliddin RAZZOKOV supervised the work.
The authors gratefully acknowledge the financial support from the Ministry of Higher Education, Science, and Innovations of the Republic of Uzbekistan (Nos. AL-4821012320 and AL-5921122141).
[1] |
Sathya K et al 2022 Appl. Water Sci. 12 70 doi: 10.1007/s13201-022-01594-7
|
[2] |
Clark M 2011 Handbook of textile and industrial dyeing: principles, processes and types of dyes (Cambridge: Woodhead Publishing
|
[3] |
Bashir I et al 2020 Concerns and Threats of Contamination on Aquatic Ecosystems In: Hakeem K R, Bhat R A and Qadri H Bioremediation and Biotechnology: Sustainable Approaches to Pollution Degradation Cham: Springer 2020: 1 doi: 10.1007/978-3-030-35691-0_1
|
[4] |
Daniel Enns et al 2023 Water Res. 243 120388 doi: 10.1016/j.watres.2023.120388
|
[5] |
Ahmed M et al 2022 npj Clean Water 5 12 doi: 10.1038/s41545-022-00154-5
|
[6] |
Manish Kumar et al 2023 Environ. Pollut. 320 121009 doi: 10.1016/j.envpol.2023.121009
|
[7] |
Fakhri B et al 2024 Sustain. Environ. 10 2322831 doi: 10.1080/27658511.2024.2322821
|
[8] |
Zhou S et al 2024 Water Sci. Tech. 89 1539 doi: 10.2166/wst.2024.040
|
[9] |
Alsukaibi A K D 2022 Processes 10 1968 doi: 10.3390/pr10101968
|
[10] |
Donkadokula N Y, Kola A K and Saroj D 2020 Sustain. Environ. Res. 30 9 doi: 10.1186/s42834-020-00050-y
|
[11] |
Kesari K K et al 2021 Water Air Soil Pollut. 232 208 doi: 10.1007/s11270-021-05154-8
|
[12] |
Garrido-Cardenas J A et al 2019 Int. J. Environ. Res. Public Health. 25 17 doi: 10.3390/ijerph17010170
|
[13] |
Lucas M S, Peres J A and Li Puma G 2021 Water 13 1309 doi: 10.3390/w13091309
|
[14] |
Chen Y D et al 2021 Chem. Eng. J. 409 128207 doi: 10.1016/j.cej.2020.128207
|
[15] |
Saravanan A et al 2022 Chemosphere 308 136524 doi: 10.1016/j.chemosphere.2022.136524
|
[16] |
Litter M I and Quici N 2010 Recent Patents Eng. 4 217 doi: 10.2174/187221210794578574
|
[17] |
El-Gawad H A et al 2023 Sci. Rep. 13 3420 doi: 10.1038/s41598-023-29263-y
|
[18] |
Arifin M N et al 2023 Environ. Res. 229 115936 doi: 10.1016/j.envres.2023.115936
|
[19] |
Suwannarat S et al 2024 ACS Appl. Bio. Mater. 7 1469 doi: 10.1021/acsabm.3c00862
|
[20] |
Adamovich I et al 2017 J. Phys. D: Appl. Phys. 50 323001 doi: 10.1088/1361-6463/aa76f5
|
[21] |
Nishijima A et al 2019 Mod. Plast. Surg. 9 18 doi: 10.4236/mps.2019.91004
|
[22] |
Domonkos M et al 2021 Appl. Sci. 11 4809 doi: 10.3390/app11114809
|
[23] |
Christos A 2022 Chem. Eng. J. 428 131657 doi: 10.1016/j.cej.2021.131657
|
[24] |
Cyganowski P et al 2023 Plasma Chem. Plasma Process. 43 199 doi: 10.1007/s11090-022-10285-9
|
[25] |
Locke B R et al 2006 Ind. Eng. Chem. Res. 45 882 doi: 10.1021/ie050981u
|
[26] |
Motyka A et al 2018 Biotechnology and Bioengineering. 115 1581 doi: 10.1002/bit.26565
|
[27] |
Upadrasta A et al 2023 Journal of Applied Microbiology. 134 lxad181 doi: 10.1093/jambio/lxad181
|
[28] |
Malik M A 2010 Plasma Chem. Plasma Process. 30 21 doi: 10.1007/s11090-009-9202-2
|
[29] |
Morin-Crini N et al 2022 Environ. Chem. Lett. 20 1333 doi: 10.1007/s10311-021-01379-5
|
[30] |
Tao X M et al 2016 J. Environ. Manage. 184 480 doi: 10.1016/j.jenvman.2016.10.038
|
[31] |
Kumar A et al 2021 Eur. Phys. J. D 75 283 doi: 10.1140/epjd/s10053-021-00283-5
|
[32] |
Takeuchi N and Yasuoka K 2020 Jpn. J. Appl. Phys. 60 SA0801 doi: 10.35848/1347-4065/abb75d
|
[33] |
Yin Y et al 2023 Appli. Sci. 13 12631 doi: 10.3390/app132312631
|
[34] |
Rahimpour M et al 2019 J. Environ. Chem. Eng. 7 103220 doi: 10.1016/j.jece.2019.103220
|
[35] |
Abdelmalek F et al 2006 Ind. Eng. Chem. Res. 45 23 doi: 10.1021/ie050058s
|
[36] |
Sun B et al 2012 J. Environ. Sci. 24 840 doi: 10.1016/S1001-0742(11)60837-1
|
[37] |
Magureanu M et al 2008 Plasma Chem. Plasma Process. 28 677 doi: 10.1007/s11090-008-9155-x
|
[38] |
Zeghioud H et al 2020 J. Water Process Eng. 38 101664 doi: 10.1016/j.jwpe.2020.101664
|
[39] |
Laroussi Mounir et al 2022 IEEE Trans. Radiat. Plasma Med. Sci. 6 127 doi: 10.1109/TRPMS.2021.3135118
|
[40] |
Kooshki S et al 2024 J. Water Process Eng. 63 105477 doi: 10.1016/j.jwpe.2024.105477
|
[41] |
Hefny M M et al 2016 J. Phy. D: Appl. Phy. 49 404002 doi: 10.1088/0022-3727/49/40/404002
|
[42] |
Benedikt J et al 2018 Phys. Chem. Chem. Phy. 20 12037 doi: 10.1039/C8CP00197A
|
[43] |
Lin P 2020 J. Phy. D: Appl. Phy. 54 075205 doi: 10.1088/1361-6463/abc2f1
|
[44] |
Zhang Y T, Gao S H and Ai F 2023 Front. Physics. 11 1125548 doi: 10.3389/fphy.2023.1125548
|
[45] |
Elaissi S and Alsaif N A M 2023 Polymers 15 1235 doi: 10.3390/polym15051235
|
[46] |
Elstner M et al 1998 Phys. Rev. B 58 7260 doi: 10.1103/PhysRevB.58.7260
|
[47] |
Gaus M, Goez A and Elstner M 2013 J. Chem. Theory and Comput. 9 338 doi: 10.1021/ct300849w
|
[48] |
Elstner M, Frauenheim T and Sándor S 2003 J. Mol. Struct.: THEOCHEM. 632 29 doi: 10.1016/S0166-1280(03)00286-0
|
[49] |
Yusupov M et al 2023 Plasma Processes Polym. 20 2200137 doi: 10.1002/ppap.202200137
|
[50] |
Xu S F, Jirasek V and Lukes P 2020 J. Phys. D: Appl. Phys. 53 275204 doi: 10.1088/1361-6463/ab8321
|
[51] |
Dabagh A et al 2023 Heliyon 9 e21977 doi: 10.1016/j.heliyon.2023.e21977
|
[52] |
Kandjani G O et al 2023 Plasma Process. Polym. 20 e2200192 doi: 10.1002/ppap.202370008
|
[53] |
del Pilar Carreón-Castro, M. et al 2010 Thin solid films 518 4136 doi: 10.1016/j.tsf.2009.11.066
|
[54] |
Guo X Y et al 2021 Chin. J. Org. Chem. 41 1703 doi: 10.6023/cjoc202010033
|
[55] |
Fernandes F H, Bustos-Obregon E and Salvadori D M F 2015 Reprod Toxicol. 53 75 doi: 10.1016/j.reprotox.2015.04.002
|
[56] |
da Silva Leite L et al 2016 Chemosphere 148 511 doi: 10.1016/j.chemosphere.2016.01.053
|
[57] |
Porezag D et al 1995 Phys. Rev. B. 51 12947 doi: 10.1103/PhysRevB.51.12947
|
[58] |
Seifert G, Porezag D, and Frauenheim T 1996 Int. J. Quantum Chem. 58 185 doi: 10.1002/(SICI)1097-461X(1996)58:2<185::AID-QUA7>3.0.CO;2-U
|
[59] |
Hourahine B et al 2020 J. Chem. Phys. 31 152 doi: 10.1063/1.5143190
|
[60] |
Qian H J et al 2011 J. Chem. Theory Comput. 7 2040 doi: 10.1021/ct200197v
|
[61] |
Gaus M et al 2014 J. Chem. Theory Comput. 10 1518 doi: 10.1021/ct401002w
|
[62] |
Kubillus M et al 2015 J. Chem. Theory Comput. 11 332 doi: 10.1021/ct5009137
|
[63] |
Saha B et al 2009 ACS Nano. 3 2241 doi: 10.1021/nn900494s
|
[64] |
Aradi B, Hourahine B and Frauenheim T 2007 J. Phys. Chem. A 111 5678 doi: 10.1021/jp070186p
|
Number | Event | Frequency | Details | |
1 | H-abstraction | C8−OH | 3 | Formation of hydroxyl group |
2 | C8−OH | 7 | ||
3 | H-abstraction | C11−OH | 8 | |
4 | C11−OH | 1 | Formation of hydroxyl group | |
5 | C11O1−OH | 3 | Formation of hydroperoxide group | |
6 | C11 and O1H; H2O | 3 | Formation of aldehyde group | |
7 | H-abstraction | C14−OH | 16 | Formation of hydroxyl group, see figure 4 |
8 | H-abstraction | C9−OH | 1 | Formation of hydroxyl group |
9 | C9−OH | 1 | ||
10 | H-abstraction | C12−OH | 5 | |
11 | C13−OH | 3 | Formation of hydroxyl group | |
12 | C15−OH | 2 | ||
13 | C16−OH | 2 | ||
14 | H-abstraction | C19−OH | 1 | |
15 | C22−OH | 3 | Formation of hydroxyl group | |
16 | C23−OH | 1 | ||
17 | H-abstraction | H2O and CH2O | 12 | Formation of water molecule and formaldehyde (see figure 5) |
18 | O-addition | N6−O | 3 | Addition of oxygen to the azo group (see figure 6(a)) → may lead to loss of color |
19 | N5−O | 3 | ||
20 | O-addition | C20−O−C23 | 3 | Addition of oxygen to the benzene ring (see figure 6(b)) |
21 | C16−O−C17 | 1 | Ring-expansion | |
22 | C21−O−C22 | 2 | ||
23 | C10−O−C13 | 1 | ||
24 | C13−O−C16 | 1 | ||
25 | C10−O−C12 | 1 | ||
26 | C18−O−C20 | 3 | ||
27 | O-addition | — | 5 | Benzene ring-opening (see figure 6(c)) |
28 | O-addition | C19−O | 1 | Addition of oxygen to C19, detachment of H connected to C19 and binding of it to N5, formation of ketone group |
29 | O-addition | N7O3−O | 2 | |
30 | C21=O and NO2 | 1 | Formation of C21=O bond, dissociation of C21−N7 bond and subsequent detachment of NO2 from the system | |
31 | O-addition | N4+O | 1 | |
Total number of events | 100 |