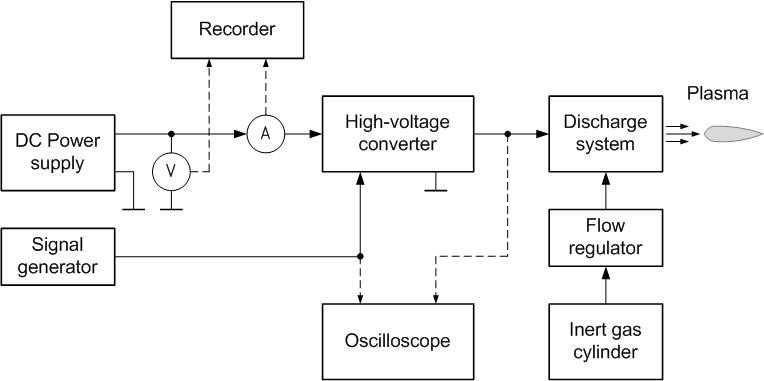
Citation: | Tianbao MA, Yauheni KALENKOVICH, Valeriy ROKACH, Anatoly OSIPOV. Generation of low-temperature plasma by pulse-width modulated signals and monitoring of the interaction thereof with the surface of objects[J]. Plasma Science and Technology, 2025, 27(1): 015403. DOI: 10.1088/2058-6272/ad8a38 |
The article discusses the use of pulse-width modulation signals to generate low-temperature atmospheric plasma in an inert gas environment. The results of studies of the energy consumption of a low-temperature plasma generation system depending on the duty rate, as well as the pulse repetition rate, are presented. The operating modes of the system have been established, in which a minimum of energy consumption is achieved. The issues of evaluating the interaction of plasma with objects based on the analysis of changes in signal parameters in the high-voltage circuit of the generator are also considered.
The current stage of society’s development is characterized by the rapid introduction of new promising technologies that allow solving a wide range of tasks in various fields of science and technology. One of these technologies is a technology based on the use of low-temperature atmospheric plasma [1–4]. The scope of application of this technology is quite extensive, the main most important areas are microelectronics (product manufacturing), optics (processing and coating), chemical industry and the production of new materials (changing the surface properties of various materials in a non-destructive manner), environmental protection (water, air purification technologies, etc.), biology and healthcare (equipment and technologies of sterilization and therapy) and others. The advantages of processing in low-temperature plasma at atmospheric pressure are the absence of the need for bulky systems for building and maintaining a vacuum, the ability to work with various kinds of materials, including low-temperature polymers and biological objects, low cost of equipment in comparison with vacuum-plasma equipment, mobility and versatility of application.
One of the directions for improving generators of low-temperature atmospheric plasma is the use of special design, technological and circuit solutions that reduce overall dimensions, power consumption, ensure the stability of the operating modes of generators, etc.. In currently manufactured plasma generation devices, a high-voltage sinusoidal voltage is applied to the discharge system. The issues of using other form signals for these purposes are poorly understood. In modern electronic equipment, signals with pulse-width modulation (PWM) are widely used to control the power supplied to the load [5, 6]. In addition, the widespread use of low-temperature atmospheric plasma technologies is hampered by the lack of simple methods to control its interaction with objects, especially with biological objects. Especially when plasma is excited by non-sinusoidal signals. In this regard, this paper discusses the use of PWM-based signals for the generation of low-temperature atmospheric plasma and an effective way to control the interaction of plasma with the surface of objects based on the analysis of the dynamics of changes in the output electrical power.
To conduct experimental studies, a laboratory research stand was used, the block diagram of which is shown in figure 1.
The research stand includes the following units: a DC power supply, a high-voltage converter, a signal generator, a discharge system, a gas flow regulator, an inert gas cylinder, a voltage and current parameter recorder with appropriate meters for determining power consumption and an oscilloscope. The system for generating high-voltage alternating voltage (up to 4 kV) includes a DC power supply with the ability to adjust voltage and current respectively in the range from 0 to 30 V and from 0 to 10 A, respectively, a high-voltage converter and a signal generator capable of generating a pulse-width modulated signal with the adjustable parameters.
The standard signal generator OWON AG 4151 was used as a PWM pulse generator. The high-voltage converter is based on a flyback pulse converter and consists of a high-voltage pulse transformer, a transistor switch and protection elements. The voltage transformation coefficient of the transformer is 1:300.
The direct formation of plasma is carried out in a coaxial discharge system, through which gas is passed and a plasma torch is formed at the outlet [7]. A coaxial-type discharge unit with dielectric barrier discharge is used as a discharge system to create a diffuse-type plasma at atmospheric pressure. This discharge unit allows forming a plasma plume up to 3 cm long, with a treatment zone with a diameter of about 1 cm.
Argon (18Ar) is used as a plasma-forming gas. The amount of gas entering the discharge system is adjusted using a gas flow regulator. For all conducted experiments, the condition of constant volume of inert gas consumption of 25–26 L/h was fulfilled.
To control operation modes current and voltage recorder and digital oscilloscope are used. Since one channel of the oscilloscope is connected to the high-voltage part of the circuit, a 1:1000 high-voltage voltage divider was used to register the high-voltage signal.
It is known [8] that the step-up transformer, electrodes and other elements of the discharge system together with the generated plasma form an oscillatory circuit, which has resonant properties. In this regard, in this work, studies of plasma generation depending on the time-frequency parameters of the PWM signal supplied to the discharge system are carried out. At the same time, the experiments were carried out under conditions of mandatory plasma combustion without taking into account its quantitative and qualitative characteristics and properties (luminance, dimensions and temperature of the torch, etc.).
Thus, at the first stage of the experiments, the effect of the duty cycle of PWM control pulses on the energy consumption of a plasma formation device at a resonant frequency fr=1/T was studied. The high-voltage converter was supplied with control pulses of a rectangular shape with a fixed voltage amplitude from the signal generator (figure 2(a)). The duty cycle D of the PWM signal (the ratio of the pulse width to the pulse period in percent, D=(τ/T)⋅100%) decreased from 50% (half of the oscillation period at the resonance frequency) to the value at which the visible burning of the plasma stopped.
At the second stage, the operation of the plasma formation device was investigated when the repetition period of the master pulses did not coincide with the period of the self-resonance of the formed circuit (figure 2(b)). At the same time, the duration of the master pulse remained unchanged and equal to τ=1/(2fr), i.e. half of the oscillation period at the resonant frequency. The repetition rate of the PWM signal master pulses varied from the value of the resonant frequency (≈ 48.8 kHz) downwards.
The parameters of voltage and current consumption were recorded with the help of the recorder, and the formed signals on the discharge system electrodes were observed with the help of a digital oscilloscope.
Low-temperature atmospheric pressure plasma interacting with the surface of an object is a load for a high-voltage alternating current source in a circuit with a periodic signal source. A change in the nature of interaction with the surface of the object is accompanied by a change in the load impedance and, accordingly, a change in the signal parameters (instantaneous values of voltages and currents) exciting the plasma, as well as the power released on the load. In this regard, the technique used in this work to evaluate the interaction of plasma with the surface of an object is based on measuring the parameters of the signals exciting the plasma (instantaneous values of voltage and currents), calculating and analyzing changes in power (instantaneous and average values over a period) released on a plasma-object load [9].
Thus, at the third stage, precision measurement of the values of instantaneous voltages and currents on the electrodes of the discharge unit of the plasma generator was performed on a real time scale. The determination of voltage and current values is carried out by means of a photometric method developed by the authors, which provides a galvanic isolation of the high-voltage circuit of the plasma generator from the measuring part with the necessary measurement accuracy [10]. The recorded parameters of the electrical signal causing plasma generation were transmitted to the computing device through an external analog to digital converter (ADC) module. In accordance with the known expressions [11], the instantaneous reactive Q(n), true P(n) and apparent power S(n) allocated to the load were calculated and the corresponding dependencies were constructed, where n is the reference number proportional to time t. Next, the average values of active, reactive and apparent power are calculated during the period under study.
When applying control pulses to the output stage from a signal generator with a duty cycle of 50% (tortuous), undamped sinusoidal oscillations will be observed on the secondary winding of a high-voltage transformer (on the electrodes of the discharge system) (figure 3(a)). A decrease in the duty cycle of the PWM signal leads to a decrease in the voltage of the observed signal Ue on the electrodes, as shown in the figure 3(b). For the waveforms shown in the figure, when the duty cycle was reduced to 25%, the signal amplitude decreased by 40%.
Figure 4 shows graphs of the dependence of the power consumed by the generator on the duty cycle of the PWM signal at the resonance frequency at different supply voltages. As can be seen from the graphs presented, a decrease in the duty cycle leads to a decrease in power consumption. When a certain duty cycle of the PWM signal was reached, the plasma torch went out, and the discharge glow in the discharge system was not observed. For supply voltages of 18, 15 and 12 V, the minimum duty cycle, at which plasma luminescence was still observed, was 7%, 9% and 14%, respectively.
Three sections should be highlighted on the graphs, characterizing the different operating modes of the device in terms of energy consumption. In section 1, the power consumed by the generator decreases with a decrease in the duty cycle D. In the second section, the power practically does not change. For example, when the supply voltage E is 15 V and duty cycle PWM signal is 26%, the ionized noble gas density reaches the highest value and does not change to value signal duty cycle 45%. It means that in the pulse width range from 26% to 45%, the ionized noble gas density is the same. In the third section, there is also a decrease in power consumption with a decrease in the duty cycle D.
During this research, the temperature at the center of the plasma plume, measured by the contact method, was between 35 °C and 40 °C. A decrease in the D factor resulted in a decrease in the cold plasma plume temperature in the specified range. Thus, changing the pulse duration at the resonant frequency supplied to the high-voltage converter makes it possible to operate the generator in various power consumption modes.
The pulse duration of the PWM signal determines the energy transferred to the inert gas atoms for the transition to the ionized state, and is determined by the parameters of the output oscillating circuit (the transformation coefficient of a high-voltage transformer, the parameters of the magnetic permeability of the core, the Q-factor of the oscillating circuit, etc.).
It is known that when a single rectangular pulse is applied to the input of an oscillatory circuit in a resonant system, damped oscillations occur with a frequency equal to the resonant frequency fr. When a periodic sequence of master pulses is applied (figure 2(b)) with a duty cycle of less than 50%, periodically damped oscillations occur on the electrodes of the discharge system (figure 5). The duration of the periodically damped oscillation depends on the Q-factor of the resonant system Q and can be determined using the logarithmic decrement of attenuation λ [12]. For the system under study, the measured logarithmic decay decrement was λ = 0.65.
The ratio of the duration of the pulse τ and its period of repetition TG, which determines the moment when the pulse energy enters the circuit in the presence of freely damped oscillations, significantly affects the efficiency of using PWM signals for plasma generation [13]. Thus, figure 6 shows the waveforms of the pulses with different values of the repetition period and the corresponding voltage waveforms on the electrodes of the discharge system. The duration of the pulse remained constant and equal to τ=1/(2fr). In figures 6(a) and (b) and during the periods of repetition of the pulses, respectively, TG1=1fr, TG2=2⋅1fr and TG3=4⋅1fr are equal. The number of periods of freely damped oscillations for these cases is 0, 1 and 3, respectively. At the same time, the amplitude of the voltage of the damped oscillation at the time of the arrival of a new pulse is zero. That is, the final phase of the damped oscillations and the initial phase of the driving pulses coincide. This ensures the maximum initial voltage amplitude of the excited damped oscillations.
It follows from the time diagram shown in figure 6(d) that, depending on the frequency of the master pulses, the initial phase of a new oscillation may not coincide with the final phase of a freely damped oscillation. This leads to the fact that part of the energy of the incoming pulses is spent on recharging the reactive elements of the output oscillatory circuit and leads to additional energy costs.
Therefore, the use of PWM signals with parameters τ=1/(2fr) can also ensure a reduction in energy costs for plasma generation when working with a period of repetition of the master pulses longer than when working at the resonance frequency of the output circuit of the discharge system. In addition, it should be noted that stable plasma combustion is ensured by increasing the pulse repetition period to a certain value TG=TGmax (figure 7). This critical (maximum) period of the master pulses is determined by the maximum amplitude (level) Usmin of the last period of damped oscillations, at which the energy is sufficient to excite the plasma, and depends on the attenuation decrement. The greater the attenuation decrement λ, the faster the energy stored in the circuit is consumed and, therefore, less TGmax.
Figure 8 shows the dependence of the power consumption on the repetition frequency of the PWM signal master pulses at E = 18VDC and τ=1/(2fr) = const. The frequency of the PWM signal varied from 1 (duty cycle is 1.02%) to 60 kHz (duty cycle is 61.5%).
As can be seen from the graph, the presented dependence has several local minima of power consumption. They are observed at the resonant frequency fr = 48.8 kHz and at frequencies equal to f1min = fr/2 = 24.4 kHz, f2min = fr/3 = 16.3 kHz, f3min = fr/4 = 12.2 kHz and f4min = fr/5 = 9.76 kHz. At these frequencies of local minima of power consumption fimin (i = 1, 2, 3 and 4), the initial phase of the master pulses coincides with the final phase of periodically damped oscillations. The number of periods of freely damped oscillations (excluding the first period of forced oscillation) corresponds to fr/fi−1=k−1. The frequencies fi can be used as the operating frequencies of the plasma generator. The maximum number n is determined from the condition of the presence of plasma. As follows from the graph, plasma generation at frequencies fi leads to a decrease in power consumption, while, with a decrease in the values of frequenciesfi, a decrease in the values of power consumption is observed. Thus, the use of the frequency f3min = 12.2 kHz for plasma generation instead of the resonance frequency fr = 48.8 kHz led to a decrease power consumption by 29%.
In addition to local minima of power consumption, there are also local maxima of energy consumption at frequencies f1max = 36.6 kHz, f2max = 20.3 kHz, f3max = 14.2 kHz, f4max =10.98 kHz and f5max = 9 kHz. This is explained by the fact that at these frequencies, the phase difference between a freely damped oscillation and the beginning of a forced one is π. The mismatch of the oscillation phases leads to additional losses in the output circuit and an increase in power consumption by the generator. Plasma generation at frequencies above the resonance frequency fr is characterized by an increase in the duty cycle D of more than 50% and, accordingly, leads to an increase in power consumption.
To determine the effective frequency band of the PWM master pulses, which provide energy-efficient operating modes near the fundamental resonance frequency fr and for frequencies of local minimum of power consumption fimin, we use the well-known definition of the bandwidth of the oscillatory circuit [12]:
Δfimin=fr⋅λ2π⋅k. | (1) |
Then the conditions for selecting the frequency of the PWM signal are determined from:
{fi=frk, k=1,2,…;fi−fr⋅λ4π⋅k⩽ | (2) |
When choosing the frequency {f}_{i\mathrm{m}\mathrm{i}\mathrm{n}} of the local minimum power consumption, with an increase in the number of periods of freely damped oscillations (with an increase in k ), a decrease in the corresponding effective frequency band is observed.
Thus, the use of damped voltage on the electrodes of the discharge system, formed on the basis of PWM signals, allows controlling the operating modes of the plasma generator.
To process the results of the measured instantaneous voltage and current values in the output circuit of the plasma generator and evaluate its interaction with objects, a program has been developed in the Matlab environment. The program works as follows. The instantaneous values of voltage U(n) and current I(n) (figures 9(a) and (b)) are stored in the computing device. The amplitude values of voltage and current are indicated in relative units.
The studied sequence contains 9 studied (repetitive) signal periods. However, due to the instability of the frequency of the pulse generator {f}_{\mathrm{G}} , the number of complete periods in the recorded sequence may be less than one, that is M = 8. Accordingly, the sampling frequency of the {f}_{\mathrm{s}} signals determines the duration of the processed signal {T}_{0} , as well as the number of processed periods of the plasma excitation signal M=N\cdot \dfrac{{f}_{\mathrm{G}}}{{f}_{\mathrm{s}}}-1 .
Next, instantaneous electrical power is calculated in accordance with the well-known expression [5]. The graph of the dependence of the change in instantaneous power for the signals shown in figure 9 is shown in figure 10.
The recorded current and voltage signals shown in figures 9 and 10 have a periodic damped character. As can be seen from the figures, when interacting with an object, there is a phase shift indicating that the system ceases to operate in resonance. This is shown by the presence of a negative component in figure 11.
As follows from figures 9 and 10, the processed sequences contain 8 complete repeating periods. The selection of the analyzed periods of the studied sequences is performed based on the search for local extremes. For further processing, the dependence of instantaneous voltages U(n), current I(n), and power P(n) can be used in the program. However, more clearly the negative extremes Pmin i and the corresponding time counts nmin i are characteristic of the dependence P(n), where i = 0,…, M is the sequence number of the local extremum starting from zero. The first full period begins with the countdown of time nmin i, corresponds to the local negative extremum Pmin i, and the last one ends at time nmin 8, which determines the location of Pmin 8.
The algorithm for selecting extremes based on the analysis of the dependence of the change in instantaneous power is implemented as follows.
Starting from the zero value from n0 to n0 + Δn, the minimum value Pmin 0 of the first local extremum and the corresponding countdown nmin 0 are searched for. The search interval Δn is determined from the expression:
\mathrm{\Delta }n=\left(\frac{1}{{f}_{\mathrm{G}}}+\frac{1}{{{f}^{2}}_{\mathrm{G}}}\cdot \mathrm{\Delta }f\right)\cdot {f}_{\mathrm{s}} , | (3) |
where \dfrac{\Delta f}{{f}_{\mathrm{G}}} is the relative instability of the frequency of the master oscillator, \Delta f is the magnitude of the frequency departure.
Thus, the value nmin 0 corresponding to the extremum Pmin 0 is determined. Next, the search is performed for the next local extremum of the analyzed dependence Pmin 1, nmin 1 corresponding to the local extremum in the interval from nmin 1 to nmin 1 + \mathrm{\Delta }n .
The procedure for searching for local extremes Pmin i and their location nmin i is repeated over time intervals from nmin i−1 to nmin i−1 + \mathrm{\Delta }n , respectively.
Thus, for further processing, the intervals are formed: nmin 0 ÷ nmin 1, nmin 1 ÷ nmin 2 … nmin i−1 ÷ nmin i. For the selected intervals, the energy parameters are calculated – true, reactive and apparent electrical power:
{P}_{i}=\frac{1}{{n}_{\mathrm{min}\;i}-{n}_{\mathrm{m}\mathrm{i}\mathrm{n}\;i-1}}{\sum }_{{n}_{\mathrm{m}\mathrm{i}\mathrm{n}\;i-1}}^{{n}_{\mathrm{m}\mathrm{i}\mathrm{n}\;i}}U\left(n\right)I\left(n\right),\;{\mathrm{at}}\;U\left(n\right)I\left(n\right)\geqslant 0 , | (4) |
{Q}_{i}=\frac{1}{{n}_{\mathrm{m}\mathrm{i}\mathrm{n}\;i}-{n}_{\mathrm{m}\mathrm{i}\mathrm{n}\;i-1}}{\sum }_{{n}_{\mathrm{m}\mathrm{i}\mathrm{n}\;i-1}}^{{n}_{\mathrm{m}\mathrm{i}\mathrm{n}\;i}}U\left(n\right)I\left(n\right),\;{\mathrm{at}}\;U\left(n\right)I\left(n\right) < 0 , | (5) |
\left|{S} _{i}\right|=\frac{1}{{n}_{\mathrm{m}\mathrm{i}\mathrm{n}\;i}-{n}_{\mathrm{m}\mathrm{i}\mathrm{n}\;i-1}}{\sum }_{{n}_{\mathrm{m}\mathrm{i}\mathrm{n}\;i-1}}^{{n}_{\mathrm{m}\mathrm{i}\mathrm{n}\;i}}\sqrt{{\left({P}_{i}\right)}^{2}+{\left({Q}_{i}\right)}^{2}} . | (6) |
In accordance with the obtained values of the active, reactive and apparent power of signals, the dependence Pi, Qi and Si are constructed. Figure 11 shows the dependence of the change in the apparent power released at the load when the distance from the plasma torch to the object changes. A plastic plate was used as the object. Figure 12 shows the dependence of changes in apparent, reactive and true power when interacting with various objects.
A significant increase in the total power (by 21%) is observed when the distance between the flare and the surface is increased from 2.0 to 2.5 cm. When the distance is further increased, the power values remain almost constant.
As can be seen from the graphs, the total measured power did not change significantly when different objects interacted with the surface. However, the active power for the saline cuvette exceeded the corresponding power for the human plasma cuvette. For reactive power, this difference is reversed.
Cuvettes with various fillings (saline and human plasma) were used as objects. As follows from the presented figures, when plasma interacts with objects, the energy characteristics of the signals generating plasma change. The average values of true, reactive and apparent power in relative units for the period under review, respectively, were as follows: when interacting with a cuvette with saline solution, S00 = 243.87, P00 = 32.35, Q00 = −243.477, when interacting with a cuvette with human plasma S0 = 215.63, P0 = 40.97, Q0 = −211.613. Thus, changes in energy parameters are characteristic when changing the types of objects (the material from which the object is made, the shape of the object, etc), as well as the distance to the object.
Thus, in this paper, the use of PWM signals for excitation of low-temperature atmospheric plasma is investigated. The power consumed by the plasma generator at the resonant frequency largely depends on the choice of the duty cycle value. There is a range of values of the duty cycle D, which is characterized by a constant power consumption. The remaining ranges are characterized by a decrease in power consumption with a decrease in D. When using PWM signals with a duty cycle of less than 50% and a frequency less than resonant, periodic damped oscillations occur on the electrodes of the discharge system. The dependence of power consumption on the frequency of the PWM signal is characterized by the presence of local minimum and maximum. The relations for choosing the frequency of repetition of signals from buses are proposed and justified, which provide energy-efficient modes of operation of the plasma generator and can be used to control its operation.
The issues of evaluating the interaction of plasma with objects based on the analysis of changes in signal parameters in the high-voltage circuit of the generator are considered. As a result of the study, it was found that when plasma interacts with objects, the apparent, true and reactive powers released on the plasma-object load change. It is established that the characteristics of true and reactive power have pronounced differences when changing the distance to the object, as well as changing the material of the object. This research result can be used to develop new methods for controlling the interaction of low-temperature atmospheric plasma with objects.
[1] |
Reema et al 2022 Front. Phys. 10 942952 doi: 10.3389/fphy.2022.942952
|
[2] |
Von Woedtke T, Metelmann H R and Weltmann K D 2014 Contrib. Plasma Phys. 54 104 doi: 10.1002/ctpp.201310068
|
[3] |
Kumar A et al 2021 Eur. Phys. J. D 75 283 doi: 10.1140/epjd/s10053-021-00283-5
|
[4] |
Adamovich I et al 2022 J. Phys. D: Appl. Phys. 55 373001 doi: 10.1088/1361-6463/ac5e1c
|
[5] |
Itshoki Y S and Ovchinnikov N I 1972 Pulse and digital devices, “Soviet Radio”, p 592 (In Russian
|
[6] |
Mek R 2008 Switching power supplies, “Theoretical foundations of design and a guide to practical application”, p272 (In Russian
|
[7] |
Kang H R et al 2017 IEEE Trans. Plasma Sci. 45 691 doi: 10.1109/TPS.2017.2678527
|
[8] |
Su C F et al 2021 Electronics 10 2119 doi: 10.3390/electronics10172119
|
[9] |
Osipov A N and Kotov D A 2023 Method of diagnostics of dielectric barrier discharge plasma (Date of issue 05.12.2023), Eurasian Patent for invention No. 045560, Russian Federation (In Russian
|
[10] |
Osipov A N 2023 Device for measuring current in a high-voltage circuit (Date of issue 12.01.2023), Eurasian Patent for invention No. 042096, Russian Federation (In Russian
|
[11] |
Bladyko Y V 2009 Electrical engineering and electronics p 90. (In Russian
|
[12] |
Gonorovsky I S 2006 Radio engineering circuits and signals Moscow: Drofa, p 719 (In Russian
|
[13] |
Osipov A N, Kotov D A and Kalenkovich E N 2023 Method of generating dielectric barrier discharge plasma (Date of issue 29.05.2023), Eurasian Patent for invention No. 043498, Russian Federation (In Russian
|
[1] | Zelong ZHANG (张泽龙), Jie SHEN (沈洁), Cheng CHENG (程诚), Zimu XU (许子牧), Weidong XIA (夏维东). Generation of reactive species in atmospheric pressure dielectric barrier discharge with liquid water[J]. Plasma Science and Technology, 2018, 20(4): 44009-044009. DOI: 10.1088/2058-6272/aaa437 |
[2] | Xingmin SHI (石兴民), Jinren LIU (刘进仁), Guimin XU (许桂敏), Yueming WU (吴月明), Lingge GAO (高菱鸽), Xiaoyan LI (李晓艳), Yang YANG (杨阳), Guanjun ZHANG (张冠军). Effect of low-temperature plasma on the degradation of omethoate residue and quality of apple and spinach[J]. Plasma Science and Technology, 2018, 20(4): 44004-044004. DOI: 10.1088/2058-6272/aa9b78 |
[3] | Zhigang LI (李志刚), Zhongcai YUAN (袁忠才), Jiachun WANG (汪家春), Jiaming SHI (时家明). Simulation of propagation of the HPM in the low-pressure argon plasma[J]. Plasma Science and Technology, 2018, 20(2): 25401-025401. DOI: 10.1088/2058-6272/aa93f8 |
[4] | Zhuang LI (李壮), Xiuling ZHANG (张秀玲), Yuzhuo ZHANG (张玉卓), Dongzhi DUAN (段栋之), Lanbo DI (底兰波). Hydrogen cold plasma for synthesizing Pd/C catalysts: the effect of support–metal ion interaction[J]. Plasma Science and Technology, 2018, 20(1): 14016-014016. DOI: 10.1088/2058-6272/aa7f27 |
[5] | Yuyang WANG (汪宇扬), Cheng CHENG (程诚), Peng GAO (高鹏), Shaopeng LI (李少鹏), Jie SHEN (沈洁), Yan LAN (兰彦), Yongqiang YU (余永强), Paul K CHU (朱剑豪). Cold atmospheric-pressure air plasma treatment of C6 glioma cells: effects of reactive oxygen species in the medium produced by the plasma on cell death[J]. Plasma Science and Technology, 2017, 19(2): 25503-025503. DOI: 10.1088/2058-6272/19/2/025503 |
[6] | Zengchao JI (季曾超), Shixiu CHEN (陈仕修), Shen GAO (高深). Mechanism analysis of radiation generated by the beam-plasma interaction in a vacuum diode[J]. Plasma Science and Technology, 2017, 19(1): 15003-015003. DOI: 10.1088/1009-0630/19/1/015003 |
[7] | Jianxun LIU (刘建勋), Yanyun MA (马燕云), Xiaohu YANG (杨晓虎), Jun ZHAO (赵军), Tongpu YU (余同普), Fuqiu SHAO (邵福球), Hongbin ZHUO (卓红斌), Longfei GAN (甘龙飞), Guobo ZHANG (张国博), Yuan ZHAO (赵媛), Jingkang YANG (杨靖康). High-energy-density electron beam generation in ultra intense laser-plasma interaction[J]. Plasma Science and Technology, 2017, 19(1): 15001-015001. DOI: 10.1088/1009-0630/19/1/015001 |
[8] | WANG Guibin (王桂滨), ZHANG Lin (张林), HE Feng (何锋), OUYANG Jiting (欧阳吉庭). Numerical Study on Microwave Scattering by Various Plasma Objects[J]. Plasma Science and Technology, 2016, 18(8): 791-797. DOI: 10.1088/1009-0630/18/8/01 |
[9] | DI Lanbo (底兰波), ZHAN Zhibin (詹志彬), ZHANG Xiuling (张秀玲), QI Bin (亓滨), XU Weijie (徐伟杰). Atmospheric-Pressure DBD Cold Plasma for Preparation of High Active Au/P25 Catalysts for Low-Temperature CO Oxidation[J]. Plasma Science and Technology, 2016, 18(5): 544-548. DOI: 10.1088/1009-0630/18/5/17 |
[10] | DING Liang (丁亮), HUO Wenqing (霍文青), YANG Xinjie (杨新杰), XU Yuemin (徐跃民). The Interaction of C-Band Microwaves with Large Plasma Sheets[J]. Plasma Science and Technology, 2012, 14(1): 9-13. DOI: 10.1088/1009-0630/14/1/03 |