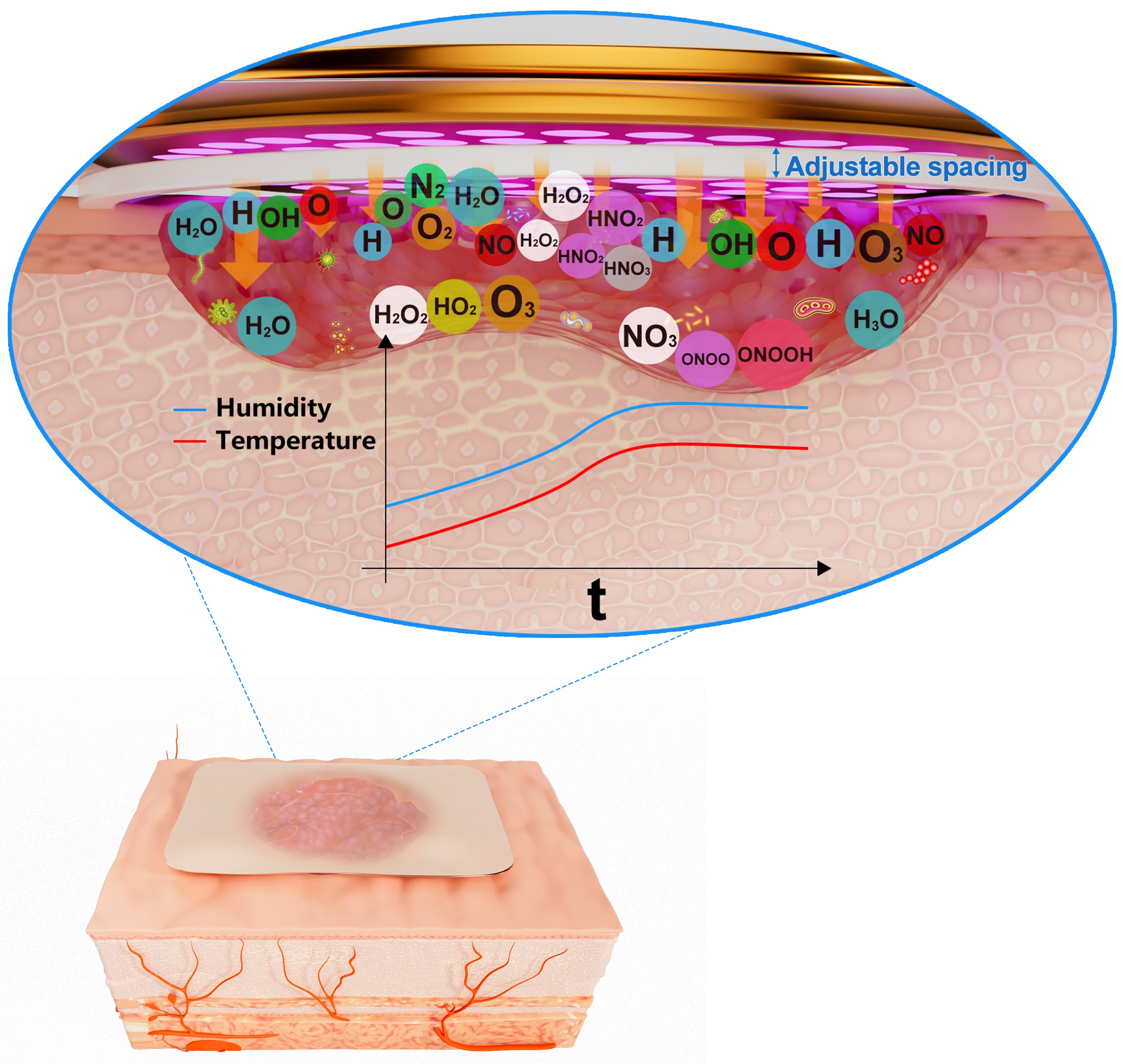
Citation: | Jingyun ZHANG, Min ZHU, Chaohai ZHANG. Dynamic of mode transition in air surface micro-discharge plasma: reactive species in confined space[J]. Plasma Science and Technology, 2025, 27(1): 015402. DOI: 10.1088/2058-6272/ad862c |
Flexible surface micro-discharge plasma is a non-thermal plasma technique used for treating wounds in a painless way, with significant efficacy for chronic or hard-to-heal wounds. In this study, a confined space was designed to simulate wound conditions, with gelatin used to simulate wound tissue. The distinction between open and confined spaces was explored, and the effects of temperature, humidity, discharge power and the gap size within the confined space on the plasma characteristics were analyzed. It was found that temperature, humidity and discharge power are important factors that affect the concentration distribution of active components and the mode transition between ozone and nitrogen oxides. Compared to open space, the concentration of ozone in confined space was relatively lower, which facilitated the formation of nitrogen oxides. In open space, the discharge was dominated by ozone initially. As the temperature, humidity and discharge power increased, nitrogen oxides in the gas-phase products were gradually detected. In confined space, nitrogen oxides can be detected at an early stage and at much higher concentrations than ozone concentration. Furthermore, as the gap of the confined space decreased, the concentration of ozone was observed to decrease while that of nitrate increased, and the rate of this concentration change was further accelerated at higher temperature and higher power. It was shown that ozone concentration decreased from 0.11 to 0.03 μmol and the nitrate concentration increased from 20.5 to 24.5 μmol when the spacing in the confined space was reduced from 5 to 1 mm, the temperature of the external discharge was controlled at 40 °C, and the discharge power was 12 W. In summary, this study reveals the formation and transformation mechanisms of active substances in air surface micro-discharge plasma within confined space, providing foundational data for its medical applications.
In recent years, surface dielectric barrier discharge (SDBD) has resulted in widespread applications in biomedicine for its operability and efficiency, generating large-area plasma in atmospheric pressure [1–6] such as chronic ulcer treatment [7], dental health [8], cancer therapy [9, 10], etc. The flexible SDBD structure, due to its high flexibility and reliability, has been extensively applied in coagulation [11, 12] and wound healing [13]. SDBD produces reactive oxygen and nitrogen species (RONS), which present strong oxidizing properties [14], enabling it to play a crucial role in plasma medicine applications.
RONS produced by plasma include reactive oxygen species (ROS) and reactive nitrogen species (RNS). ROS are highly oxidizing, inducing oxidative stress in cells, affecting bacterial morphology and disrupting cell membrane integrity [15]. RNS can be converted into strong oxidants in acidic media. It has been confirmed that RNS have important applications in the field of nitrogen fixation as well as in biomedical fields, such as tumor apoptosis and wound healing. Thus, different species have different effects. Generally, RONS exist in two forms; the one in the gas phase such as O3, O, NO, NO2 and OH [16, 17]. When the gas-phase plasma dissolves in the tissue or liquid, it is converted to the liquid-phase form, including O−2, 1O2, NO−2, NO−3, H2O2 and ONOO− [18, 19], which can be detected in plasma-activated water (PAW). The chemical characteristic of reactive species depends on environmental conditions, such as temperature and humidity, gas flow rate and other discharge conditions, which affect the chemical reaction rate. Therefore, the reactive species density and discharge mode, including the ozone mode and nitrogen oxide mode could be modulated [20–22]. Park et al [23] found that ozone was produced immediately and the concentration increased rapidly when the discharge was ignited. However, the increasing trend was reversed with increasing temperature. Compared to O3, the production of NO2 is not significant at first. At room temperature, NO is oxidized to NO2 at a fast rate and is further oxidized to the higher oxidation state N2O5. Xu et al [24] also found that the time taken to reach ozone peak concentration increased with increasing temperature. Meanwhile, it was observed that the NO2 concentration increased significantly at higher temperature, after which the concentration gradually stabilized. It has been confirmed that increased humidity promotes NO production and allows NO, NO2 and N2O5 to be deeply oxidized to HNO2 and HNO3 [25–27]. The concentrations of OH, HO2 and NH gradually increase with increasing humidity, resulting in a faster conversion of NO2 to NO3 [28].
When the flexible plasma generator is applied to human tissue, a variable and complex environment is formed in a confined and narrow space, accompanied by heat generation due to continuous discharge, as shown in figure 1. Meanwhile, the discharge conditions are also an important factor for the generation and conversion of active species, which modulates the chemical reaction properties of RONS. However, there are few reports on the generation and conversion mechanisms of RONS in a confined space. Most studies focus on a single factor such as discharge power or the confined space gap.
Shimizu et al [29] found that mode transition occurred when the air surface discharge in a confined space was higher than 0.1 W cm−2 and the ozone density started to decrease. In recent years, the air gap in the confined space between electrode and liquid region has received extensive attention. Numerical models of gas plasma in contact with liquid have been reported for physicochemical processes in plasma [30], and it is found that different particles have a dependence on the time and air gap spacing, especially when the air gap increases. Furthermore, the spatial distributions of reactive species were quantified for the air gap width ranging from 0.1 to 2 cm, the concentration of reactive species increased with the increase in the air gap from 0.1 to 0.5 cm and then decreased with the higher gap [31]. Similar trends were revealed by Li et al [32] for both the ‘open’ and the ‘closed’ volume for different distances between the electrode and bacteria surface. They found the optimum distance for achieving the highest bactericidal efficiency. However, how RONS can be correlated to the ‘closed’ volume is not well understood, nor are the trends with complex environmental conditions such as temperature or humidity. Therefore, it is necessary to study the effect of discharge conditions and confined-space state on the generation of reactive oxygen and nitrogen species, which is significant to exploit clinically practical SDBD-based biomedical device technology.
In this study, the main objectives are to systematically analyze the effect of temperature and relative humidity (RH) on the plasma electrical characteristics and the concentration characteristics of reactive species, and try to determine the correlation between open and confined spaces. The reactive species generated by plasma are assessed by measuring them in the gas phase and liquid phase, using Fourier transform infrared spectroscopy and ultraviolet spectrophotometry, respectively. This will contribute to the modulation of plasma chemical reactions and the development of clinically effective patch-type medical device technology based on flexible surface dielectric barrier discharge.
A schematic diagram of the experimental set up is shown in figure 2. The experiments were conducted in an air atmosphere (T = 20 °C, RH = 55%). Figure 2(a) shows the side view of the SDBD structure. The flexible surface dielectric barrier discharge plasma generator consists of a flat high-voltage copper electrode, a grounded mesh copper electrode, and an insulating dielectric layer sandwiched between the two electrodes, based on a printed circuit board (PCB) bonded together. The electrodes are 35 μm thick and the size of the disc is 50 mm in diameter. The dielectric layer is a polyimide sheet (50 μm thick), which can be bent at will to achieve a tight fit and ensure uniform plasma coverage. Figure 2(b) shows the gas-phase system and figure 2(c) shows the liquid-phase system. The chamber is a 10 cm × 10 cm × 7 cm cube made of 5 mm thick acrylic plate. The reaction chamber as well as the plasma generator are placed in a chamber with adjustable temperature and humidity. Considering that the flexible plasma generator involved herein acts on human skin tissue or with wounds, it is not suitable for the temperature parameter to be too high and for the humidity to be too low. Therefore, a temperature range of 20 °C–40 °C and a humidity range of 50%–80% are chosen.
The high-voltage electrode is connected to an AC pulse modulated power supply with a modulating pulse frequency of 200 Hz, a modulating pulse duty cycle of 40% and a pulse repetition frequency of 2 kHz. The discharge power can be calculated by integrating the applied voltage and current [33, 34]. The peak-to-peak voltage was measured with a high-voltage probe (Tektronix, P6015A) and the current was measured with a current probe (Pearson 6585). Their waveforms were recorded by an oscilloscope (Tektronix MDO3034). The breakdown voltage was acquired by taking images of the mesh surface discharge and current waveforms. Discharge power can be given by:
Pd=1τ∫τ0V(t)I(t)dt, | (1) |
where τ is the period of the applied voltage, and V(t) and I(t) are the instantaneous values of the applied voltage and current, respectively.
The infrared temperature distribution on the surface of the mesh electrodes was obtained by an infrared imager (Fluke Thermography Ti32) and the distance between the infrared lens and the mesh electrodes was fixed. At the same time, it is important to ensure the same baseline temperature before each test, which means the same inception temperature of the electrode surface and chamber. To detect gas-phase products, two gas paths were installed in the system. One was connected to a Fourier transform infrared spectrometer (FTIR, Thermo Fisher Scientific) to analyze the reaction products in the wavenumber range of 500–2500 cm−1. The other gas path was connected to an ozone analyzer to detect ozone concentration.
For the liquid-phase RONS detection system, human tissue models made of gelatin gels with a diameter of 6 cm and a thickness of 1 mm are used. Gelatin gels were prepared by mixing pure gelatin powders (MP biomedicals) with water solution according to standard protocols. The mass fraction of water in human skin is usually 70%, which corresponds to a gelatin mass fraction of 30% [35]. The gasket was placed between the mesh electrode and the gelatin. The mesh electrode, gasket and gelatin model were stacked in the center, aligned to form a confined space. The plasma generator produces the active substance in the confined space and diffuses it into the gelatin, and it finally penetrates the PAW. The experimental setup is shown in figure 2(c). The height of the gasket is adjustable to change the confined spacing and modulate the plasma reactive species reaction. The active substance can be detected by spectrophotometry after plasma treatment. The absorbance of the active particles at specific wavelengths was measured using a UV spectrophotometer [36, 37]. It needs to be emphasized that the wavelengths with maximum absorption peaks have been determined by ozone standard solution, nitrate standard solution and hydrogen peroxide standard solution, respectively.
Figure 3 depicts the influence of temperature and humidity on breakdown voltage and discharge power. Figure 3(a) shows the typical voltage–current (V–I) waveform during the discharge on-time under the condition of atmospheric pressure (1 atm) and ambient environment. Figure 3(b) shows that the breakdown voltage tends to rise with increasing humidity, and with the increase in temperature, this increasing trend is significantly strengthened. However, the impact of temperature on breakdown voltage depends on the humidity. At 50% and 60% humidity, breakdown voltage decreases with increasing temperature. At 70% humidity, the breakdown voltage first decreases and then increases with increasing temperature. At 90% humidity, the breakdown voltage consistently increases with increasing temperature. Overall, the effect of humidity on breakdown voltage is singular, i.e. the breakdown voltage increases with increasing humidity. However, the effect of temperature on breakdown voltage shows different trends in different humidity ranges.
The trend of plasma discharge power variation with temperature and humidity is depicted in figure 3(c). Temperature is identified as a significant factor affecting discharge power, rather than humidity. An increase in temperature may induce a greater number of micro-discharges, thereby promoting an increase in discharge power [38]. Similar trends in power variation with humidity have also been observed in other types of discharge. Hähnel et al used SDBD [39], Chiper et al used DBD [40] and Sivachandiran and Khacef used pin-plate pulsed corona discharge [41], and they found the total discharge power in the reactor was not dependent on the humidity in the present discharge system.
To analyze the evolution of gas-phase active species concentrations in flexible SDBD under various environmental conditions, as well as different discharge powers, a controllable temperature and humidity environment was created. Figure 4 shows the concentration of O3 within 6 min of discharge with different discharge powers. It can be seen from figure 4 that the ozone concentration rises rapidly at the start of the discharge, which may be due to the diffusion of ozone by the gases in the chamber and pipeline, and then reaches its peak after 4 min of discharge. When the power is increased from 4 to 8 W, the ozone peak concentration increases with increasing power. It is worth noting that the peak concentration of O3 decreases when the power is increased from 8 to 12 W. In addition, the evolution of concentration shows a tendency to increase and then decrease when the discharge power is 10 and 12 W, respectively. At higher discharge powers, it is possible that the onset of ozone mode transition to nitrogen oxide mode is responsible for the decrease in ozone concentration.
To further explore the formation process of the ozone and nitrogen oxides in the gas phase, the reactive species under two discharge powers of 8 and 12 W within 6 min are identified by FTIR. The evolution of plasma exhaust gases at 20 °C and RH = 50% under discharge power of 8 W is shown in figure 5. It should be noted that the ozone peak concentration shows a tendency to increase over the on-discharge time. However, there are no nitrogen compound peaks detected, which means that it is an ozone-only mode. Plasma-generated ozone initiation involves electron collision-induced dissociation of oxygen molecules, listed as follows:
e+O2→e+2O | (2) |
e+N2→e+2N | (3) |
O+O2+O2→O3+O2 | (4) |
O+O2+N2→O3+N2 | (5) |
Due to the higher dissociation energy of nitrogen molecules than its excitation energy and the oxygen excitation energy, only ozone is produced under these discharge conditions.
Figure 6 presents the reactive species spectra at various temperatures and humidities under discharge power of 8 W. In figure 6(a), the spectrum is dominated by ozone with no notable nitrogen compound peaks. As the humidity increases from 50% to 70%, there is no significant change in the spectra. As the humidity increases to 80%, the ozone concentration decreases slightly. When the temperature is elevated to 30 °C in figure 6(b) with humidity at 50% and 60%, the ozone peak does not change significantly. However, as the humidity increases to 70% and 80%, there is a noticeable reduction in the ozone peak. At the same time, faint N2O peaks emerge. With a further increase in temperature to 40 °C, the ozone peak is even lower compared to the previous temperature of 30 °C, and continues to decrease as the humidity gradually increases. Increased humidity leads to more compounds of nitrogen oxide peaks, with NO2 and HNO3 peaks visible at 70% humidity. Similar trends were noted in a DBD-based reactor [42]. We believe that the ozone mode begins to diminish gradually. To investigate the characteristics and modes of active species at varying discharge power, the plasma power increases to 12 W, which significantly enhances the discharge intensity, with all other conditions remaining the same as previously described. Figure 7 shows the dynamic species spectrum at 20 °C and RH = 50%. It should be noted that the peak concentration of ozone decreases compared to figure 5. Meanwhile, a small amount of N2O and NO2 is detected, which means it is easier to produce nitrogen oxides. Nitrogen compounds appear at 2 min and different peaks can be detected at 4 min of discharge.
Figure 8 shows the formations of RONS with different temperatures and humidities at 12 W. Comparing the result of the FTIR in figure 6, it should be noted that the peak concentration of ozone decreases significantly, and the decreasing trend becomes faster with the increase in temperature and humidity. Meanwhile, more nitric oxides are produced, accompanied by an earlier time to be identified. As can be seen from figure 8, as the temperature increases from 20 °C to 40 °C, the ozone concentration is much lower, and the ozone peak at 2100 cm−1 approaches the detection threshold.
By contrast, the peaks of N2O, NO2, HNO3 and N2O5 increase monotonically as the temperature increases from 20 °C to 30 °C. In figure 8(b), the peak concentration of HNO3 increases with increasing humidity. Continuing to increase the temperature to 40 °C, the concentrations of HNO3, NO2 and N2O increase sharply with increasing humidity. NO peaks are notably absent due to its quick oxidation to NO2 and further to N2O5. NO is predominantly oxidized to NO2, and N2O5 contributes to the increased NO2 levels through decomposition [43], which accounts for the higher NO2 levels in figure 8 versus figure 6. The system is dominated by the nitrogen oxide mode. Since the reaction chamber contains a small amount of water vapor, nitrogen oxides, such as N2O, NO2, N2O5 and HNO3, are identified.
The FTIR spectra in figures 6 and 8 suggest that ozone is the key reactive specie produced by SDBD across the whole range of temperatures and humidities, and the dependency of nitrogen species on the temperature and humidity at different discharge powers is also illustrated.
It has been reported that the primary products in PAW are O3, nitrite, nitrate and H2O2 [44, 45], which is also applicable to gelatin tissues. In order to explore the factors affecting the concentration of active substances in a confined space, we consider several factors involving different discharge durations (t = 1, 2, 3, 4, 5 and 6 min), various gas gaps between plasma and gelatin tissue (H = 5, 3 and 1 mm), different plasma powers (8 and 12 W), and the temperatures of PAW (20 °C and 40 °C).
Figure 9 shows the infrared surface temperatures of the mesh electrode after 1–6 min of continuous discharge. The temperature gradually increases during the 6 min of discharge, from 22 °C after 1 min to 27.2 °C after 6 min when the discharge power is fixed at 8 W. With the same baseline temperature, the surface electrode temperature rises faster when the power is increased to 12 W. After 1 min of discharge, the infrared temperature is 25.1 °C, 3 °C higher than at 8 W, and stabilizes at 35 °C, 8 °C higher than at 8 W. The increased electrode temperature contributes to higher confined space temperature and humidity.
For the liquid-phase experiment, we also validated the selection of discharge power for different modes, as depicted in figure 10. It is observed that there is an inverse trend between ozone and nitrate concentrations with increasing power at 20 °C. The ozone concentration increases slightly as the power increases from 4 to 8 W. As the power increases from 8 to 12 W, a significant decrease in ozone concentration is observed, consistent with the gas-phase results shown in figure 4. However, the nitrate concentration increases dramatically.
Figures 11 and 12 show the time-dependent concentrations of O3, H2O2, and nitrate in PAW at various confined space gaps and plasma discharge powers, where the temperature of PAW in figure 11 is 20 °C and in figure 12 is 40 °C. Figure 11(a) shows that the ozone concentration increases gradually during the first 3 min, and then the concentration is in a steady state of dynamic equilibrium. The ozone concentration decreases significantly as the discharge power increases, and the concentration does not continue to decrease after 4 min. It also been observed that the ozone concentration changes slightly as the confined space gap decreases. For nitrate, the concentration increases over the on-discharge time and twofold over that after the power increases to 12 W, as shown in figure 11(b). Notably, the nitrate concentration increases as the confined gap decreases. It shows the more pronounced rise at higher discharge power, which indicates that the confined space gap affects nitrate concentration significantly. Compared with the results in the gas phase shown in figure 5, no peak of nitrogen compounds was detected at 20 °C and 8 W. However, nitrates can be detected in the PAW within 1 min, as shown in figure 11(b) under the same conditions.
Figure 12 shows the concentration trends for reactive species in PAW at 40 °C. For the ozone concentration in figure 12(a), the result is different from figure 11(a), with the overall concentration decreasing by 70%. When the discharge power is increased to 12 W, the ozone concentration further decreases by more than 90%, indicating a mode transition to the nitrogen oxide mode. The decrease in ozone concentration is faster with a reduced confined gap, suggesting that higher temperature and narrow space are detrimental to ozone production. It is found that the concentration of ozone in the ozone mode can be up to 15 times higher than that in the nitrogen oxide mode when the temperature of PAW rises to 40 °C. For nitrate in figure 12(b), when the temperature rises to 40 °C and the power is 8 W, the concentration is two times higher than in figure 11(b). When the power is further increased to 12 W, the concentration is seven times higher compared to figure 11(b), indicating that higher power and temperature conditions promote the mode transition to nitrogen oxide mode. For hydrogen peroxide in figures 11(c) and 12(c), the concentration increases with longer discharge time and higher power, but decreases under high-temperature conditions. It is also influenced by the confined space gap, which mainly forms from the dissolution of gas phase, resulting from water molecule dissociation in the core plasma [38]. It is found that temperature and confined spacing gap significantly regulate the concentration of active species in the plasma discharge.
As known, environmental conditions play a significant role in plasma characteristics. The electrical characteristics of plasma can be influenced by the temperature and humidity. Rigorous studies found that humidity affects breakdown voltage of discharge caused by changing adhesion coefficients on electrode and dielectric surfaces [46–49]. Nawawi et al [50, 51] investigated the effect of humidity on discharge inception voltage. He found a large decline in the surface resistivity with increasing relative humidity and therefore, diminished the charge accumulation. Centurioni et al [52] confirmed that due to the electronegativity of water, the accumulated charge on the electrode surface is lower at higher humidity level, which caused the inception voltage to increase. A similar trend was found by Fu et al when they studied the recovery of water from high-temperature wet flue gas. They also attributed this variation to the combination of water molecules and free electrons [53]. In low humidity environments, temperature primarily affects the breakdown voltage. In our case, at 70% humidity, the humidity effect in the air is enhanced. The enhanced effect of humidity is further intensified as the temperature increases, causing the breakdown voltage to increase. Go [54] stated that the ionization reaction rate increases with rising temperature, and the generated electrons and ions facilitate the development of streamer discharge. In this study, the breakdown voltage increases gradually with increasing humidity. Temperature presents a more significant effect on the discharge power compared to humidity.
The reactive species, including ozone and nitrogen oxides, are significantly influenced by temperature, humidity, and power in terms of the types and concentrations. Humidity promotes the relaxation rates of N2 and O2 in plasma from vibrational to transformational energy [33]. That is to say, the high humidity has negative effects on electron accumulations, leading to fewer O atoms from electron impact dissociation of oxygen molecules, and thus reduces ozone production. The addition of H2O results in more water-based radicals, such as OH, HO2, and NH that generates NO, enhancing NO2 production [55]. NO and NO2 are further oxidized to higher valence nitrogen oxides:
e+H2O→H+OH+e | (6) |
O(1D)+H2O→2OH | (7) |
N+OH→NO+H | (8) |
N(2D)+H2O→OH+HN | (9) |
HN+O2→NO+OH | (10) |
NO+O+M→M+NO2 | (11) |
NO+HO2→NO2+OH | (12) |
NO+OH→N2O+H | (13) |
N(2D)+NO→N2O | (14) |
N+NO2→N2O+O | (15) |
O(3P)+N2+NO2→NO3+N2 | (16) |
NO3+NO2+N2→N2O5+N2 | (17) |
Gaseous NO and NO2 react with water to form nitrate and nitrite products:
NO+OH→HNO2 | (18) |
NO2+OH→HNO3 | (19) |
NO+H2O2→HNO2+OH | (20) |
NO2+H2O2→HNO3+OH | (21) |
We did not detect HNO2 peaks in the FTIR spectra, possibly due to the low concentration below the detection threshold or its quick decomposition into NO and OH by plasma UV radiation [56]. In addition, ozone concentration decreases with increasing temperature because higher temperatures accelerate ozone decomposition, at the same time enhancing the formation of nitrogen oxides such as NO2 and N2O. This is attributed to more intense molecular motion and energetic electron collisions at higher temperatures, which excite nitrogen to form NO, leading to O3 quenching and NO oxidation to NO2 via the following reactions:
O3→O2+O | (22) |
O+O3→O2 | (23) |
N2(A)+O→NO+N | (24) |
NO+O3→NO2+O2 | (25) |
It is demonstrated that the discharge system is dominated by the ozone mode under low temperature and humidity. In high-temperature and high-humidity environments, the system transitions to the nitrogen oxide mode. Increasing plasma power results in greater electron density, initially enhancing ozone production. However, the rise in the reduced electric field increases average electron energy, promoting nitrogen molecule excitation and dissociation, leading to more N atoms and N2(A). These species can form NO, which is further oxidized to NO2, NO3 and N2O5. The resulting products compete with ozone formation reactions, suppressing ozone generation and initiating a transition mode in SDBD. All the reaction rates of the above reactions change with increasing the temperature, as shown in figure S1 in the supplementary data. As a result, the number and type of the formed species can also change. The temperature dependence of the rate constants for the main particle chemical reactions in this study was acquired from the National Institute of Standards and Technology (NIST), which is summarized in figure S1 of the supplementary data.
N+O2→O+NO | (26) |
N+O3→O2+NO | (27) |
N2(A3Σ+u)+O2→N2O+O | (28) |
N2(A3Σ+u)+O2→N2O+O(1D) | (29) |
N2O5+O→NO2+NO2+O2 | (30) |
This study introduces the application of flexible plasma patches on simulated skin tissue to analyze the factors affecting reactive species concentrations. Confined space conditions are modulated via the temperature, confined spacing gap and discharge power. The shorter confined space gap more markedly affects plasma chemical reactions. On one hand, the survival period of short-life particles is generally less than 1 ms, contributing to the short diffusion distance. On the other hand, the shorter the confined area, the lower the background gas content, which cannot even meet the needs of redox reactions of all active particles. Therefore, the concentration of reactive species is strongly dependent on the gas area below the discharge electrode. The ozone production rate may diminish over the on-discharge time due to oxygen and nitrogen consumption. Abdelaziz et al also illustrated that the energy yield of ozone increases with increasing the oxygen content [57].
A widely accepted explanation of nitrate concentration increasing with reduced confined space gap is the higher Henry’s law constant compared to ozone and hydrogen peroxide [34]. In addition, the oxygen content in the space influences the transformation rates of reactive species, as reported by Teodoru et al [55]. The concentration of nitrate maintains a dynamic equilibrium after the peak concentration, without significant decline, which may be attributed to the consistently humid conditions that favor NO formation. It can be interpreted that humidity has a positive effect on nitrate formation across the entire range of oxygen content. It is more likely that the rates of production and conversion of reactive substances are regulated in two ways. The one is the space gas content, where both oxygen and nitrogen are regulated, which is important for the formation of ozone and nitrogen oxides. The other is that different active substances have significant abilities to dissolve in water. The conversion relationships of the active substances under different conditions are summarized in figure 13.
Compared to open space, the concentration of ozone detected from PAW in confined space is relatively lower due to the complex conditions and its characteristics [58]. HNO3 rapidly hydrolyzes to form H+, NO−2 and NO−3, instantly detectable in PAW after discharge. Despite the lower gas-phase density, hydrogen peroxide dissolves more readily than ozone in PAW, leading to higher concentration in the liquid-phase system. The concentration characteristics of reactive species in both gas and liquid phases represent similar trends under the examined experimental conditions, although differences exist such as variations in spatial distribution and detectability. In high-temperature and high-humidity environments, particularly with high power, an extended discharge time further accelerates the rate of concentration change, expediting the formation and conversion of reactive species in confined spaces. This study contributes to the regulation of reactive species in patch-type medical device technology based on flexible surface dielectric barrier discharge.
This study systematically analyzes the effect of temperature, humidity, discharge power as well as the gap of confined space on the concentration characteristics of reactive species and the mode transition between the ozone mode and nitrogen oxide mode. Diverse measurement techniques are employed to try to determine the correlation between the open and confined space. The main findings are summarized as follows:
(1) For the open space, temperature and humidity play a significant role in plasma electrical characteristics such as the breakdown voltage and discharge power. The ozone concentration increases with discharge but decreases with increasing temperature and humidity, especially at higher discharge power, whereas these conditions promote the production of nitrogen compounds.
(2) For the confined space, when flexible plasma patches are placed on simulated skin, temperature, humidity and discharge power are important factors that affect the mode transition between ozone and nitrogen oxides. Compared to open spaces, the concentration of ozone is relatively lower in confined spaces, while the formation of nitrogen oxides is facilitated.
(3) The confined space gap between the discharge electrode and simulated skin shows a crucial influence. It is found that the shorter confined space has a positive effect on nitrate production. Moreover, the temperature of PAW also has a significant effect on the concentrations of RONS. Higher temperature and higher discharge power lead to lower ozone production and higher nitrate production, which also aligns with more rapid concentration change.
This study was supported by Postgraduate Research & Practice Innovation Program of Jiangsu Province (No. 1003-016001).
[1] |
Aboubakr H A et al 2018 PLoS One 13 e0194618 doi: 10.1371/journal.pone.0194618
|
[2] |
Bekeschus S et al 2020 IEEE Trans. Radiat. Plasma Med. Sci. 4 391 doi: 10.1109/TRPMS.2020.3002658
|
[3] |
Szili E J et al 2021 Appl. Phys. Lett. 119 054104 doi: 10.1063/5.0062787
|
[4] |
Laroussi M, Lu X and Keidar M 2017 J. Appl. Phys. 122 020901 doi: 10.1063/1.4993710
|
[5] |
Filipić A et al 2020 Trends Biotechnol. 38 1278 doi: 10.1016/j.tibtech.2020.04.003
|
[6] |
Zhang L Y et al 2022 J. Hazard. Mater. 435 129075 doi: 10.1016/j.jhazmat.2022.129075
|
[7] |
Fridman G et al 2008 Plasma Process. Polym. 5 503 doi: 10.1002/ppap.200700154
|
[8] |
Sladek R E J et al 2004 IEEE Trans. Plasma Sci. 32 1540 doi: 10.1109/TPS.2004.832636
|
[9] |
Zhong S Y et al 2016 Br. J. Dermatol. 174 542 doi: 10.1111/bjd.14236
|
[10] |
Adachi T 2022 Thermal Med. 38 1 doi: 10.3191/thermalmed.38.1
|
[11] |
Williamson D A, Carter G P and Howden B P 2017 Clin. Microbiol. Rev. 30 827 doi: 10.1128/CMR.00112-16
|
[12] |
Ratovitski E A et al 2014 Plasma Process. Polym. 11 1128 doi: 10.1002/ppap.201400071
|
[13] |
Boekema B K H L et al 2016 J. Phys. D: Appl. Phys. 49 044001 doi: 10.1088/0022-3727/49/4/044001
|
[14] |
Wu K Y et al 2022 Plasma Sci. Technol. 24 055405 doi: 10.1088/2058-6272/ac48e1
|
[15] |
Zhang Q et al 2013 Appl. Phys. Lett. 102 203701 doi: 10.1063/1.4807133
|
[16] |
Riès D et al 2014 J. Phys. D: Appl. Phys. 47 275401 doi: 10.1088/0022-3727/47/27/275401
|
[17] |
Graves D B 2014 Plasma Process. Polym. 11 1120 doi: 10.1002/ppap.201400068
|
[18] |
Gorbanev Y, O'Connell D and Chechik V 2016 Chemistry 22 3496 doi: 10.1002/chem.201503771
|
[19] |
Lu X et al 2016 Phys. Rep. 630 1 doi: 10.1016/j.physrep.2016.03.003
|
[20] |
Bansemer R et al 2017 Plasma Sources Sci. Technol. 26 065005 doi: 10.1088/1361-6595/aa6c34
|
[21] |
Chakraborty A et al 2023 Plasma Process. Polym. 20 2370013 doi: 10.1002/ppap.202370013
|
[22] |
Liu K, Hu Y and Lei J 2017 Phys. Plasmas 24 103513 doi: 10.1063/1.5004423
|
[23] |
Park S, Choe W and Jo C 2018 Chem. Eng. J. 352 1014 doi: 10.1016/j.cej.2018.07.039
|
[24] |
Xu H et al 2022 Plasma Sources Sci. Technol. 31 115010 doi: 10.1088/1361-6595/ac9d63
|
[25] |
Abdelaziz A A and Kim H H 2020 J. Phys. D: Appl. Phys. 53 114001 doi: 10.1088/1361-6463/ab5c78
|
[26] |
Malik M A et al 2016 Chem. Eng. J. 283 631 doi: 10.1016/j.cej.2015.07.092
|
[27] |
Tang X L et al 2018 Plasma Chem. Plasma Process. 38 485 doi: 10.1007/s11090-018-9876-4
|
[28] |
Soloshenko I A et al 2009 Plasma Sources Sci. Technol. 18 045019 doi: 10.1088/0963-0252/18/4/045019
|
[29] |
Shimizu T et al 2012 New J. Phys. 14 103028 doi: 10.1088/1367-2630/14/10/103028
|
[30] |
Liu Z C et al 2015 J. Phys. D: Appl. Phys. 48 495201 doi: 10.1088/0022-3727/48/49/495201
|
[31] |
Liu D X et al 2016 Sci. Rep. 6 23737 doi: 10.1038/srep23737
|
[32] |
Li Y F, Zimmermann J L and Morfill G E 2012 New J. Phys. 14 023058 doi: 10.1088/1367-2630/14/2/023058
|
[33] |
Abdelaziz A A, Ishijima T and Seto T 2018 Phys. Plasmas 25 043512 doi: 10.1063/1.5020271
|
[34] |
Xie S R et al 2019 Plasma Sci. Technol. 21 055505 doi: 10.1088/2058-6272/aafc50
|
[35] |
Davis F E, Kenyon K and Kirk J 1953 Science 118 276 doi: 10.1126/science.118.3062.276
|
[36] |
Wang B C et al 2016 IEEE Trans. Plasma Sci. 44 3295 doi: 10.1109/TPS.2016.2620565
|
[37] |
Winter J et al 2014 J. Phys. D: Appl. Phys. 47 285401 doi: 10.1088/0022-3727/47/28/285401
|
[38] |
Zeng M J, Qu Z G and Zhang J F 2023 Int. J. Heat Mass Transfer 201 123561 doi: 10.1016/j.ijheatmasstransfer.2022.123561
|
[39] |
Hähnel M, von Woedtke T and Weltmann K D 2010 Plasma Process. Polym. 7 244 doi: 10.1002/ppap.200900076
|
[40] |
Chiper A et al 2006 J. Otpoelectron. Adv. Mater. 8 208
|
[41] |
Sivachandiran L and Khacef A 2016 RSC Adv. 6 29983 doi: 10.1039/C6RA02972H
|
[42] |
Matsui K, Ikenaga N and Sakudo N 2015 Jpn. J. Appl. Phys. 54 06GD02 doi: 10.7567/JJAP.54.06GD02
|
[43] |
Li M et al 2018 Vacuum 157 249 doi: 10.1016/j.vacuum.2018.08.058
|
[44] |
Ma T P et al 2016 Plasma Sci. Technol. 18 686 doi: 10.1088/1009-0630/18/6/17
|
[45] |
Wang Z F et al 2022 Plasma Sources Sci. Technol. 31 05LT01 doi: 10.1088/1361-6595/ac60c0
|
[46] |
Meng X B et al 2015 IEEE Trans. Dielect. Electr. Insul. 22 1193 doi: 10.1109/TDEI.2015.7076822
|
[47] |
Moonesan M S, Jayaram S and Cherney E 2015 Effect of Temperature and Humidity on Surface Discharge Activities under High Voltage Unipolar Pulses In: Proc. ESA Annual Meeting on Electrostatics 2015: 1–7
|
[48] |
Wu D J et al 2020 Effect of humidity on corona characteristics under rod-plane gap In: Proceedings of the 21st International Symposium on High Voltage Engineering Cham: Springer 2020: 359
|
[49] |
Ji Y T et al 2023 IET Sci. Meas. Technol. 17 37 doi: 10.1049/smt2.12128
|
[50] |
Nawawi Z et al 2003 Effect of humidity on partial discharge characteristics In: Proceedings of the 7th International Conference on Properties and Applications of Dielectric Materials Nagoya, Japan: IEEE 2003: 307
|
[51] |
Nawawi Z et al 2006 Effect of humidity on time lag of partial discharge in insulation-gap-insulation system In: 2006 IEEE 8th International Conference on Properties & applications of Dielectric Materials Bali, Indonesia: IEEE 2006: 199
|
[52] |
Centurioni L, Guastavino F and Torello E 2002 An investigation about the PD degradation of thin polymer films and its correlation with surface charge decay measurements In: Conference Record of the 2002 IEEE International Symposium on Electrical Insulation Boston, MA, USA: IEEE 2002: 371
|
[53] |
Fu H et al 2022 Separations 9 169 doi: 10.3390/separations9070169
|
[54] |
Go D B 2022 Ionization and Ion Transport: A Primer for the Study of Gas Discharges and Plasmas 2nd ed (Bristol, UK: IOP Publishing
|
[55] |
Teodoru S, Kusano Y and Bogaerts A 2012 Plasma Process. Polym. 9 652 doi: 10.1002/ppap.201100187
|
[56] |
Yan K et al 1999 Plasma Chem. Plasma Process. 19 421 doi: 10.1023/A:1021824504271
|
[57] |
Abdelaziz A A et al 2019 Plasma Chem. Plasma Process. 39 165 doi: 10.1007/s11090-018-9942-y
|
[58] |
Seinfeld J H 1986 Environ. Sci. Technol. 20 863 doi: 10.1021/es00151a602
|
[1] | Rui LIU (刘蕊), Zhe YU (俞哲), Huijuan CAO (曹慧娟), Pu LIU (刘璞), Zhitao ZHANG (张芝涛). Characteristics of DBD micro-discharge at different pressure and its effect on the performance of oxygen plasma reactor[J]. Plasma Science and Technology, 2019, 21(5): 54001-054001. DOI: 10.1088/2058-6272/aafbbc |
[2] | Yang CAO (曹洋), Guangzhou QU (屈广周), Tengfei LI (李腾飞), Nan JIANG (姜楠), Tiecheng WANG (王铁成). Review on reactive species in water treatment using electrical discharge plasma: formation, measurement, mechanisms and mass transfer[J]. Plasma Science and Technology, 2018, 20(10): 103001. DOI: 10.1088/2058-6272/aacff4 |
[3] | Yuchuan QIN (秦豫川), Shulou QIAN (钱树楼), Cheng WANG (王城), Weidong XIA (夏维东). Effects of nitrogen on ozone synthesis in packed-bed dielectric barrier discharge[J]. Plasma Science and Technology, 2018, 20(9): 95501-095501. DOI: 10.1088/2058-6272/aac203 |
[4] | Zelong ZHANG (张泽龙), Jie SHEN (沈洁), Cheng CHENG (程诚), Zimu XU (许子牧), Weidong XIA (夏维东). Generation of reactive species in atmospheric pressure dielectric barrier discharge with liquid water[J]. Plasma Science and Technology, 2018, 20(4): 44009-044009. DOI: 10.1088/2058-6272/aaa437 |
[5] | Yunfeng HAN (韩云峰), Shaoyang WEN (温少扬), Hongwei TANG (汤红卫), Xianhu WANG (王贤湖), Chongshan ZHONG (仲崇山). Influences of frequency on nitrogen fixation of dielectric barrier discharge in air[J]. Plasma Science and Technology, 2018, 20(1): 14001-014001. DOI: 10.1088/2058-6272/aa947a |
[6] | Feng LIU (刘峰), Bo ZHANG (张波), Zhi FANG (方志), Wenchun WANG (王文春). Generation of reactive atomic species of positive pulsed corona discharges in wetted atmospheric flows of nitrogen and oxygen[J]. Plasma Science and Technology, 2017, 19(6): 64008-064008. DOI: 10.1088/2058-6272/aa632f |
[7] | Yuyang WANG (汪宇扬), Cheng CHENG (程诚), Peng GAO (高鹏), Shaopeng LI (李少鹏), Jie SHEN (沈洁), Yan LAN (兰彦), Yongqiang YU (余永强), Paul K CHU (朱剑豪). Cold atmospheric-pressure air plasma treatment of C6 glioma cells: effects of reactive oxygen species in the medium produced by the plasma on cell death[J]. Plasma Science and Technology, 2017, 19(2): 25503-025503. DOI: 10.1088/2058-6272/19/2/025503 |
[8] | JIANG Kai (蒋锴), WANG Xianrong (王先荣), QIN Xiaogang (秦晓刚), YANG Shengsheng (杨生胜), YANG Wei (杨威), ZHAO Chengxuan (赵, 呈()选 ), CHEN Yifeng (陈益峰), SHI Liang (史亮), TANG Daotan (汤道坦), XIE Kan (谢侃). Surface Charging Controlling of the Chinese Space Station with Hollow Cathode Plasma Contactor[J]. Plasma Science and Technology, 2016, 18(7): 727-731. DOI: 10.1088/1009-0630/18/7/05 |
[9] | V. PRYSIAZHNYI. Plasma Treatment of Aluminum Using a Surface Barrier Discharge Operated in Air and Nitrogen: Parameter Optimization and Related Effects[J]. Plasma Science and Technology, 2013, 15(8): 794-799. DOI: 10.1088/1009-0630/15/8/15 |
[10] | Xu Jinzhou(徐金洲), Zhong Ping(钟平), Li Jialing(李嘉灵), Ling Jie (林捷), Diao Ying(刁颖), Zhang Jing(张菁). Characteristics of Coaxial Dielectric Barrier Discharge at an Atmospheric Pressure with a Swirling Gas Argon/Oxygen Mixture for the Surface Modification of Polyester Fiber Cord[J]. Plasma Science and Technology, 2010, 12(5): 601-607. |