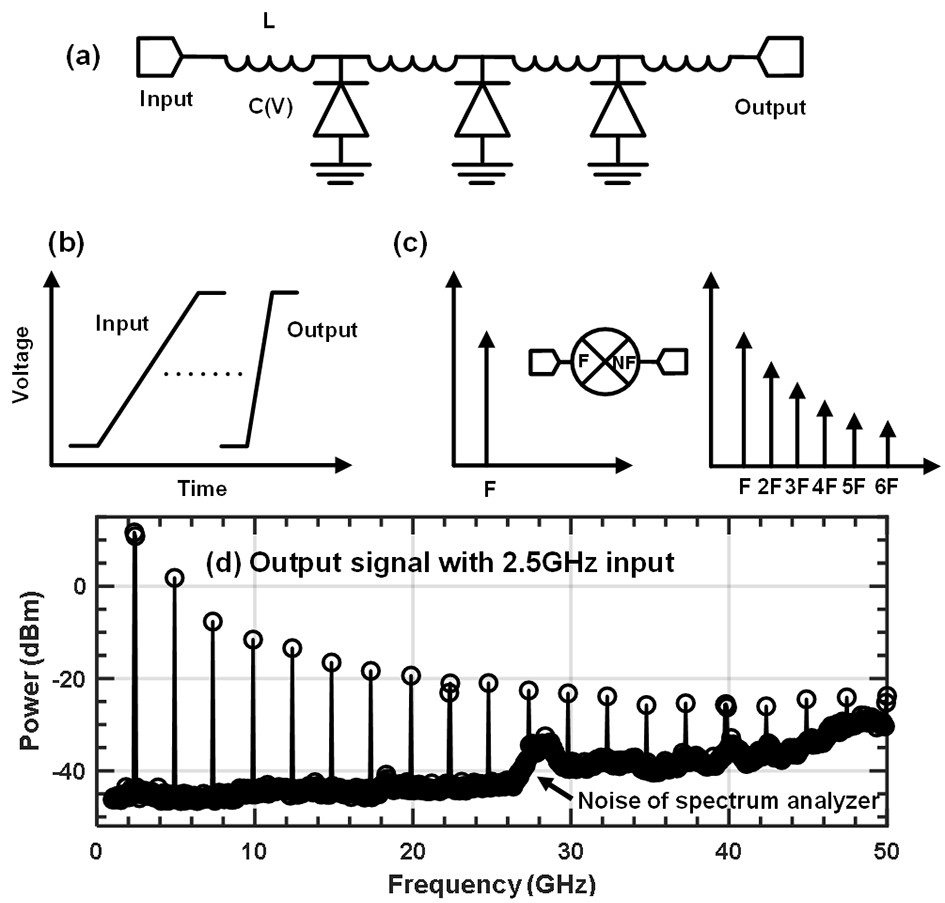
Citation: | Ruihai TONG, Yu ZHOU, Wulyu ZHONG, Jie WEN, Zhongbing SHI, Xiaolan ZOU, Anshu LIANG, Zengchen YANG, Min JIANG, Xin YU, Yuqi SHEN. A new Q-band comb-based multi-channel microwave Doppler backward scattering diagnostic developed on the HL-3 tokamak[J]. Plasma Science and Technology, 2025, 27(1): 015102. DOI: 10.1088/2058-6272/ad8c86 |
The Doppler backscattering (DBS) diagnostic is widely used to measure the localized density fluctuations and the propagation velocity of turbulent structures. Microwave is launched at a frequency that approaches a cutoff layer in the plasma at an angle oblique to the cutoff layer. A new Q-band multichannel DBS system based on a comb generator has been designed and tested for application on the HL-3 tokamak. With the comb generator and heterodyne scheme, the stability and flexibility of the new DBS system are improved. The new DBS diagnostic has a high output power (~ 10 dBm), good power flatness (< 5 dB in Q-band), and frequency stability, and the inter-frequency separation is tunable remotely. This article introduces the system design, laboratory test results, and initial experimental results from the HL-3 tokamak. With the help of the newly developed multichannel DBS, the turbulence information can be studied with high temporal and spatial resolution.
In recent years, the Doppler backscattering diagnostic has been shown to be a powerful tool for turbulence study, which can measure the local turbulence flow velocity, fluctuation levels of density turbulence, and radial correlation lengths [1, 2]. It is similar to conventional reflectometry; the major difference is to launch a microwave at an oblique angle to the cutoff layer, and the scattered signal will be refracted backward by the turbulence as the cutoff layer is approached. The Bragg condition (k⊥=−2ki) and electric field swelling effect near the cutoff make DBS a localized measurement. The Doppler shift frequency (fD) of the received signal is directly proportional to the perpendicular rotation velocity of the turbulence moving with the plasma, {u}_{\perp }={2{\text{π}}f}_{\mathrm{D}}/{k}_{\perp }={v}_{{\boldsymbol{E}}\times {\boldsymbol{B}}}+{v}_{\mathrm{p}\mathrm{h}\mathrm{a}\mathrm{s}\mathrm{e}} . Assuming the phase velocity of the turbulence ( {v}_{\mathrm{p}\mathrm{h}\mathrm{a}\mathrm{s}\mathrm{e}} ) is negligible, the plasma \boldsymbol{E}\times \boldsymbol{B} velocity can be estimated, and thence the radial electric field {E}_{r} [1, 3, 4]. The DBS diagnostic can also be used to measure the tilt angle of turbulent structures [5, 6] and magnetohydrodynamic (MHD) activity like tearing modes [7]. It has been widely developed on different fusion devices, including tokamaks [7–12], mirrors [13], stellarators [14, 15], and spherical tokamaks [16–18]. The DBS diagnostic is also planned for the ITER tokamak [19].
To obtain turbulence information with high temporal and spatial resolution, continuous multi-frequency measurement is one of the development directions of the DBS diagnostic, which has been studied and developed in various ways [8, 10–13, 15, 20–27]. One flexible way is to combine multiple independent low-frequency synthesizers and multiplier pairs for each channel and then transmit/receive with antennas [28–30]. In ASDEX Upgrade [23] and TCV [24], multi-frequency microwaves are achieved by feeding a two-tone or three-tone into a multiplier, which is generated by intermodulation between multi-tone signals in a diode. In HL-2A, a multiple fixed-frequency array source is achieved by a filter-based feedback loop [20], which is further upgraded with a multiplexer technique [12, 31]. It shows a high transmit power and good power flatness (< 5 dB). However, the output frequency of the system is not very stable during operation, which is due to temperature changes caused by amplifier operation and the use of narrowband filters in the system. The comb generator is first used on DBS in DIII-D [8]. It has been further developed on NSTX [18] and LHD [21, 25, 26]. In these systems, a broad array of harmonics is first generated from a low-frequency microwave source. Then, multipliers are used to obtain signals at higher frequencies greater than 20 GHz, such as Ka-band (26–40 GHz) [15], U-band (40–60 GHz) [21], or V-band (50–75 GHz) [8]. Due to the fact that the system response of microwave components (like multiplier or amplifier) in a wide frequency range is not flat, the output power flatness of the system is poor. The typical power flatness is around 15 dB [18, 21, 25]. In DIII-D, the E-H tuner is used to optimize the power of different frequencies to ensure the output power flatness [8]. The minimal power variation is < 6 dB in the Q and V-bands.
In this paper, a new multi-channel DBS system, based on comb generator technique which allows the simultaneous monitoring of turbulent flows and fluctuation levels at distinct spatial locations is described. The rest of the paper is organized as follows. First, the comb-based multi-channel DBS system is presented in section 2, and detailed laboratory test results for the new DBS system and a discussion on power flatness and stability are presented in section 3. In section 4, experimental data is shown to illustrate the capabilities of the multi-channel DBS system and methodology. The summary is given in section 5.
The comb generator is typically called a nonlinear transmission line (NLTL) [8]. Figure 1(a) shows a simplified NLTL schematic which is characterized by the distributed L-C elements. In a nonlinear transmission line comb generator, the Schottky junction varactor diodes are usually used. The phase velocity ( {v}_{\mathrm{p}} ) of the signal propagation along the NLTL [32] is:
{v}_{\mathrm{p}}\left(V\right)=1/\sqrt{LC\left(V\right)} , | (1) |
where L is inductance and C is the capacitance, which is a function of voltage V. As an input signal propagates through the NLTL, the phase velocity of different voltage signals will be different. Hence, cascaded L-C sections of the transmission structure cause a portion of the waveform to be increasingly vertical, as shown in figure 1(b). When a signal of a fundamental frequency is fed into NLTL, a series of frequency signals of different harmonics will be generated due to the distortion, which is shown in figure 1(c). This feature of NLTL can be used to generate a series of high-frequency signals with excellent phase noise performance, and the typical output result of the comb generator with 2.5 GHz input is shown in figure 1(d).
HL-3 (previously named as HL-2M) tokamak is a newly built medium-sized copper conductor and carbon wall tokamak in China [33], which achieved the first plasma at the end of 2020 and 1 MA plasma current recently in October 2022 [34]. The goal of the HL-3 tokamak is to address key physics and technology challenges faced by ITER and future fusion reactors. To achieve this goal, a novel diagnostic tool called the multi-frequency DBS has been developed. The new system will provide insights into the rapid turbulence behavior at the edge of the plasma, particularly phenomena like the L-H transition and edge-localized modes.
Figure 2 shows a schematic diagram of the multi-channel DBS RF system based on a comb generator on HL-3 [33, 35]. It contains three parts: (1) comb source generator and RF launcher, (2) local oscillator (LO) output, and (3) RF receiver and RF output port for intermediate frequency (IF) system.
The Marki NLTL-6275 [36] is used as the comb generator of the new DBS system in the HL-3 tokamak. It is an MMIC non-linear transmission line-based passive comb generator, which is fabricated with GaAs Schottky diode-based varactors on a 2.28 \times 3.13 mm2 substrate. This NLTL can offer excellent phase noise performance over a 3–15 GHz input frequency range and with output tones beyond 85 GHz.
Two phase-locked loops (PLLs) are used: PLL-01 is used as an input signal to the comb generator with a working frequency from 1 to 3 GHz, and PLL-02 is used as a reference signal for the RF system with a working frequency from 8 to 12.5 GHz (X-band) in 1 MHz steps. Both are referenced by the same 100 MHz crystal. First, the signal generated by the PLL-01 source is passed into the passive comb generator. Then, the comb generator will generate an array of equally spaced frequencies with a slow decay in output power. For example, with {f}_{\mathrm{P}\mathrm{L}\mathrm{L}-01} = 2.5 GHz, which is the working frequency of PLL-01, the output signal of the comb generator will be 2.5, 2.5, 50 GHz, as shown in figure 1(c). The more detailed test results will be given in section 3.
Several microwave signals in the Q-band will be selected with a wide band bandpass filter (BPF). It is designed with good performance of 3 dB bandwidth from 34 to 48 GHz. The selected array of signals is then amplified with a power amplifier (PA), which has a high saturation output power of ~ 23 dBm. Then, the Q-band output signal from PA is passed through another BPF. It should be noted that the two wideband BPFs are necessary in this system; the purposes of the BPFs include selecting the signals in a specific frequency range, enabling the amplifier to output a higher power and optimizing the power flatness, avoiding the influence of spurious signals on other microwave diagnostics, etc. A 30 dB directional coupler is used to provide a small amount of power to the RF input of a reference Q-band balanced mixer; the generated LO out signal will be used as the reference signals, and the remainder is then transmitted through an antenna. Two horn antennas are used to launch the microwave into the plasma and capture the backward scattering wave from the plasma, respectively. The position and wavenumber information of the turbulence will be obtained with the help of the measurement of the density profile, EFIT data, and ray-tracing calculation [37].
The output of the PLL-02 source is quadrupled to the Q-band by a ×4 multiplier, and it will be used as the LO input of the mixers in the RF system. Then, the Q-band RF signals will be down-shifted in frequency to an IF range. The output frequency and frequency interval in the IF range can be adjusted by changing the working frequencies of PLL-01 and PLL-02. For example, if the transmit frequency is 34, 2, 48 GHz and the reference frequency is 40.5 GHz (X-band PLL-02 is set as 10.125 GHz), then the working intermediate frequencies will be 0.5, 1.5, 2.5, 3.5, 4.5, 5.5, 6.5, and 7.5 GHz.
After down-conversion of the reference and backscattered RF signals, the LO and RF output signals will then be fed into the IF system. At each of the filtered intermediate frequencies, a quadrature mixer is used to demodulate the down-shifted reflected signal with the down-shifted reference signal. In the quadrature mixer, the in-phase (I signal) and quadrature (Q signal) signals are obtained. Then the amplitude A=\sqrt{{I}^{2}+{Q}^{2}} and the phase \phi =\mathrm{a}\mathrm{t}\mathrm{a}\mathrm{n}(Q/I) of the scattered signals caused by density fluctuation can be obtained. Finally, the I/Q signals are acquired by the digitizers (2 MS/s).
The eight-channel IF system (working frequency: 0.5, 2, 3, 4.5, 5.5, 7, 8, 9.5 GHz) developed for V-band DBS on HL-2A [31], which could adjust the gain parameters of amplifiers, is modified for the new RF system. The schematic diagram of the IF system is shown in figure 3. The designed LO and RF input power of the IF system is −10 to −30 dBm and −20 to −60 dBm respectively. The IF system is equipped with two 30 dB gain low-noise amplifiers (LNAs) and an adjustable attenuator with an adjustment range of 0–30 dB and 0.5 dB step through the remote or local interface. To be able to work at different RF frequency intervals together with variable probing frequencies, the IF system needs to replace the filter or switch different filters through a switch (not shown). Other forms of IF can also be used to adapt tunable frequency RF systems, for example, a two-step frequency down-conversion technology is developed for the need of variable comb frequency distances together with variable probing frequencies in W7-X [38].
The comb generator and multi-channel DBS system are extensively tested in the laboratory. Figure 4 shows the output power and frequency array of the comb generator with different input frequencies and powers, which are measured with a spectrum analyzer. By adjusting the frequency of the input signal, the frequency of the output signal can be adjusted accordingly. Although the NLTL-6275 is typically used with 3–15 GHz input frequency range, the test results show that it can still work well with lower frequencies like 2 and 2.5 GHz. For 2 GHz input, the typical output power is around −30 dBm. When the input frequency is increased, the output power will gradually increase due to the decrease in the number of frequency points, as shown in figures 4(a)–(c). In addition, the output power is increased with higher input power and the flatness of the output power is affected by the input power. Although the output power of the comb spectrum usually decreases with the increase of harmonic order, as illustrated in figure 1(d), the power of each frequency in the Q-band frequency range has a relatively good flatness (~ 5 dB), as illustrated in figure 4.
Figure 5 shows the launching power and frequency spectra of the Q-band comb-based DBS system with (a) 2 GHz and (b) 2.5 GHz input. The dashed line is the test result of the BPF with the help of a swept frequency source and spectrum analyzer. The launching power of each frequency component is around +10 dBm. Although the output power is lower than the multiplexer-based frequency array source (MFAS)-based DBS (~ 20 dBm), which is first developed on HL-2A [12], the power level of 10 dBm is enough to meet the need of HL-3 experiments. The output power can be further increased with a higher power PA, but the cost, volume, heat output, and power supply of the system will be increased. Since the saturated input power of the receiving mixer is usually low, an excessively high output power can cause damage to the mixer, such as abnormal reflections during testing. In addition, the signal-to-noise ratio (SNR) of the DBS system depends not only on the transmitted power but also on the phase noise. The frequency stability of the comb-based DBS is much better than the MFAS system, as shown in figure 7. The phase noise is optimized to around −100 dBc/Hz at an offset of 1 kHz (typical phase noise of MFAS system is around −80 dBc/Hz at an offset of 1 kHz [12]) in the Q-band. It is important to note that it is difficult to further optimize the phase noise level of the MFAS system, while the phase noise of a comb spectrum system can be further optimized with higher performance PLL.
For multi-frequency systems, if the power of a certain frequency is too high, it may lead to a non-linear response of components such as mixers in the system, resulting in intermodulation or intermodulation distortion [39]. To ensure the stable operation of the system within the linear response region, a good power flatness is necessary. At the same time, good power flatness will also meet the needs of the acquisition system to work in a more suitable range. The power flatness of the microwave system is affected by many factors such as operating frequency and bandwidth, response of the transmission line, amplifier performance, etc. For a full-band DBS system, the typical power flatness is around 10 dB [21, 22]. As shown in figure 5, the power variation of all frequency components is < 5 dB in the Q-band. It is optimized just by adjusting the PLL output power appropriately.
To ensure that the IF system can work properly with the comb-based RF system, the LO output signals of the RF system are carefully tested. The input frequency interval is set to 2.5 GHz, and the corresponding transmission frequencies are 35, 2.5, 47.5 GHz. The local frequency of the RF system is set at 42 GHz, with intermediate frequencies at 0.5, 2, 3, 4.5, 5.5, and 7 GHz. Figure 6 shows the power and frequency spectra of the RF output signals, which will then be used as the LO input of the IF system. The six IF points and the corresponding IF frequency are marked in figure 6(a). The results show that the working LO output power varies from −20 to −10 dBm. Although there are some signals with lower amplitude, there is no stray signal in the operating frequency band (bandpass filters with 200 MHz bandwidth are used in the IF system), as shown in figure 6(b), that can satisfy the need of the IF system. The adjustable amplifier in the IF system can further optimize the power flatness to ensure that the IF system can work properly [31]. The test results show that the comb-based DBS system on HL-3 has an improvement in power flatness and phase noise compared to the DBS system developed on LHD [21].
In HL-2A, a multiple fixed-frequency array source is achieved by a filter-based feedback loop [20], which is further upgraded using a multiplexer technique [12, 31]. It shows a high transmit power and good power flatness. However, it is found that the changes in temperature, such as the temperature change introduced by the operation of the amplifier, can cause small changes in output frequency during laboratory tests. In other words, the measurement position will change slightly with temperature. In this section, it will be shown that the new comb-based system overcomes the related problems and greatly improves the system stability.
The typical laboratory test using a spectrum analyzer is limited by low time resolution and bandwidth resolution. The typical time resolution is several seconds and the resolution bandwidth (RBW) is ~ 100 kHz for the normal spectrum analyzer. To test the stability of the new DBS system, a high-performance real-time spectrum analyzer (Tektronix RSA518A [40], working frequency from 9 kHz to 18 GHz, RBW ~ 1 Hz) is used to get a high time resolution and good bandwidth resolution simultaneously. The typical test results of the LO output frequency and power of the MFAS-based DBS and comb-based DBS are shown in figure 7. The test results with the real-time spectrum show that the MFAS system has a frequency jitter with time, while the stability of the comb spectrum system is significantly improved. It should be noted that the MFAS system typically shows two types of frequency perturbations: frequency jitter (a fast frequency change with several kilohertz on the order of tens of milliseconds, as shown in figure 7) and frequency drift (a slow frequency drift with up to several tens of megahertz on the order of tens of minutes, not shown in figure 7). Due to the improved stability, the new comb-based DBS system was able to operate continuously and stably for several days without significant frequency shifts during the HL-3 experiment.
Experiments with the newly developed DBS system have been carried out on the HL-3 tokamak. Figure 8 shows the typical result of the DBS time–frequency spectrum with X-mode polarization launch/receive at different frequencies: f = 35, 2.5, 47.5 GHz. The black line in figure 8 is the Doppler shift frequency. It is obtained from the center of gravity of the Doppler spectra, {f}_{\mathrm{D}}=\sum f\cdot S(f)/\sum S(f) , where S(f) is the power spectra of the complex I/Q signals. The positive frequency denotes the ion diamagnetic direction. There are several improvements in the analysis method for extracting Doppler waves from DBS signals which are beyond the scope of this article [41]. With multi-channel DBS simultaneously measured at six different radial positions, the turbulence behavior can be observed from the edge to the core. During gas puff, the edge turbulence is suppressed and the Doppler shift frequency is decreased from edge DBS spectra, as shown in figure 8(a). The reduction of the plasma poloidal rotation is widely observed, which may be caused by the neoclassical poloidal flow damping with increased collisionality [38]. From core DBS spectra, the intensity of broadband fluctuations (from around −500 to +600 kHz) increases rapidly during the sawtooth crash, as shown in figure 8(f). In the DIII-D experiment, it is observed that the turbulence level decreases immediately after the sawtooth crash from Te signals, and a generally gradual increase of it leading to the next sawtooth crash inside q = 1 surface using DBS [42]. It should be mentioned that the density profile may change during the sawtooth collapse, and may therefore result in a change in the DBS measurement position. Due to the lack of the density profile measurement, the actual measured wavenumber and position are not clear at the moment, and a careful interpretation of the turbulence behavior on HL-3 will be done in the near future with the upgrades of the far-infrared (FIR) interferometer [43], Thomson scattering (TS) [44], and frequency modulated continuous wave reflectometer (FMCW) diagnostics.
In this work, a new multi-channel DBS system with the probing frequency in Q-band is developed and carefully tested on the HL-3 tokamak. The RF launch part of the system is based on comb generator technology which is characterized by frequency flexibility and good stability. The IF system previously developed on the HL-2A tokamak is modified and used for the new system. The laboratory test results and primary plasma results from the HL-3 tokamak demonstrate that the newly developed DBS system has good performance, including good power flatness (< 5 dB in the Q-band) and frequency stability. As a next step, the RF system working with higher frequency and the new IF system will be designed to meet the needs of the experiment on HL-3.
This work was supported by National Natural Science Foundation of China (Nos. 12105087, 12275096, and 11922503), the Joint Funds of the National Natural Science Foundation of China (No. U21A20440), and the Science and Technology Planning Project of Sichuan Province (No. 2023YFG0139).
[1] |
Conway G D et al 2004 Plasma Phys. Control. Fusion 46 951 doi: 10.1088/0741-3335/46/6/003
|
[2] |
Hirsch M et al 2001 Plasma Phys. Control. Fusion 43 1641 doi: 10.1088/0741-3335/43/12/302
|
[3] |
Pratt Q et al 2022 Plasma Phys. Control. Fusion 64 095017 doi: 10.1088/1361-6587/ac8614
|
[4] |
Conway G et al 2006 Nucl. Fusion 46 S799 doi: 10.1088/0029-5515/46/9/S15
|
[5] |
Pinzón J R et al 2019 Plasma Phys. Control. Fusion 61 105009 doi: 10.1088/1361-6587/ab394d
|
[6] |
Pinzón J R et al 2019 Nucl. Fusion 59 074002 doi: 10.1088/1741-4326/ab227e
|
[7] |
Yashin A et al 2023 Appl. Sci. 13 3430 doi: 10.3390/app13063430
|
[8] |
Peebles W A et al 2010 Rev. Sci. Instrum. 81 10D902 doi: 10.1063/1.3464266
|
[9] |
Damba J et al 2022 Rev. Sci. Instrum. 93 103549 doi: 10.1063/5.0101864
|
[10] |
Yashin A Y et al 2022 J. Inst. 17 C01023 doi: 10.1088/1748-0221/17/01/C01023
|
[11] |
Hu J Q et al 2017 Rev. Sci. Instrum. 88 073504 doi: 10.1063/1.4991855
|
[12] |
Shi Z et al 2018 Rev. Sci. Instrum. 89 10H104 doi: 10.1063/1.5035260
|
[13] |
Kohagura J et al 2022 Rev. Sci. Instrum. 93 123507 doi: 10.1063/5.0101893
|
[14] |
Happel T et al 2009 Rev. Sci. Instrum. 80 073502 doi: 10.1063/1.3160106
|
[15] |
Tokuzawa T et al 2014 Plasma Fusion Res. 9 1402149 doi: 10.1585/pfr.9.1402149
|
[16] |
Hillesheim J C et al 2015 Nucl. Fusion 55 073024 doi: 10.1088/0029-5515/55/7/073024
|
[17] |
Bulanin V V et al 2021 Rev. Sci. Instrum. 92 033539 doi: 10.1063/5.0030307
|
[18] |
Crocker N A et al 2011 Plasma Phys. Control. Fusion 53 105001 doi: 10.1088/0741-3335/53/10/105001
|
[19] |
Vayakis G et al 2006 Nucl. Fusion 46 S836 doi: 10.1088/0029-5515/46/9/S20
|
[20] |
Shi Z et al 2016 Rev. Sci. Instrum. 87 113501 doi: 10.1063/1.4966680
|
[21] |
Soga R et al 2016 J. Instrum. 11 C02009 doi: 10.1088/1748-0221/11/02/C02009
|
[22] |
Cabrera M et al 2023 Rev. Sci. Instrum. 94 083504 doi: 10.1063/5.0151271
|
[23] |
Happel T et al 2020 Plasma Sci. Technol. 22 064002 doi: 10.1088/2058-6272/ab618c
|
[24] |
Molina Cabrera P et al 2018 Rev. Sci. Instrum. 89 083503 doi: 10.1063/1.5007433
|
[25] |
Tokuzawa T et al 2022 Appl. Sci. 12 4744 doi: 10.3390/app12094744
|
[26] |
Tokuzawa T et al 2018 Rev. Sci. Instrum. 89 10H118 doi: 10.1063/1.5035118
|
[27] |
Wang M Y et al 2018 Rev. Sci. Instrum. 89 093501 doi: 10.1063/1.5033968
|
[28] |
Wu M F et al 2020 J. Instrum. 15 P12009 doi: 10.1088/1748-0221/15/12/P12009
|
[29] |
Xiang H et al 2018 Rev. Sci. Instrum. 89 10H103 doi: 10.1063/1.5035445
|
[30] |
Ren X et al 2021 Rev. Sci. Instrum. 92 033545 doi: 10.1063/5.0040915
|
[31] |
Wen J et al 2021 Rev. Sci. Instrum. 92 063513 doi: 10.1063/5.0043676
|
[32] |
Breitbarth J 2006 “Design and Characterization of Low Phase Noise Microwave Circuits”.
|
[33] |
Duan X et al 2022 Nucl. Fusion 62 042020 doi: 10.1088/1741-4326/ac3be6
|
[34] |
Zhong W et al 2024 Innovation 5 100555 doi: 10.1016/j.xinn.2023.100555
|
[35] |
Xue L et al 2019 Nucl. Fusion 60 016016 doi: 10.1088/1741-4326/ab4c65
|
[36] |
Marki Microwave. “https://markimicrowave.com” 2024.
|
[37] |
Zhou Y et al 2023 Rev. Sci. Instrum 94 013508 doi: 10.1063/5.0126970
|
[38] |
Seol J et al 2013 Phys. Plasmas 20 042504 doi: 10.1063/1.4795731
|
[39] |
Rouphael T J 2014 Wireless Receiver Architectures and Design: Antennas, RF, synthesizers, mixed signal, and digital signal processing: Academic Press, 2014
|
[40] |
Tektronix. “https://www.tek.com/en” 2024.
|
[41] |
Zhong X et al 2023 Plasma Sci. Technol 25 095104 doi: 10.1088/2058-6272/acc8ba
|
[42] |
Wang G et al 2024 Nucl. Fusion 64 066024 doi: 10.1088/1741-4326/ad4046
|
[43] |
Mou J et al 2024 Plasma Sci. Technol. 26 034013 doi: 10.1088/2058-6272/ad127a
|
[44] |
Gong S et al 2023 Plasma Sci. Technol. 25 075601 doi: 10.1088/2058-6272/acbd8d
|
[1] | A. PONOMARENKO, A. YASHIN, V. GUSEV, E. KISELEV, G. KURSKIEV, V. MINAEV, Y. PETROV, N. SAKHAROV, P. SHCHEGOLEV, E. TKACHENKO, N. ZHILTSOV. First results of turbulence investigation on Globus-M2 using radial correlation Doppler reflectometry[J]. Plasma Science and Technology, 2024, 26(10): 105101. DOI: 10.1088/2058-6272/ad5fe5 |
[2] | Weiwei FAN (范伟伟), Bowen ZHENG (郑博文), Jing CAO (曹靖), Shibiao TANG (唐世彪), Qingwei YANG (杨青蔚), Zejie YIN (阴泽杰). Development of a fast electron bremsstrahlung diagnostic system based on LYSO and silicon photomultipliers during lower hybrid current drive for tokamak[J]. Plasma Science and Technology, 2019, 21(6): 65104-065104. DOI: 10.1088/2058-6272/ab0a77 |
[3] | Zhongbing SHI (石中兵), Wulyu ZHONG (钟武律), Min JIANG (蒋敏). Progress of microwave diagnostics development on the HL-2A tokamak[J]. Plasma Science and Technology, 2018, 20(9): 94007-094007. DOI: 10.1088/2058-6272/aad27b |
[4] | Lei YE (叶磊), Xiaotao XIAO (肖小涛), Yingfeng XU (徐颖峰), Zongliang DAI (戴宗良), Shaojie WANG (王少杰). Implementation of field-aligned coordinates in a semi-Lagrangian gyrokinetic code for tokamak turbulence simulation[J]. Plasma Science and Technology, 2018, 20(7): 74008-074008. DOI: 10.1088/2058-6272/aac013 |
[5] | Lu WANG (王璐), Shuitao PENG (彭水涛), P H DIAMOND. Gyrokinetic theory of turbulent acceleration and momentum conservation in tokamak plasmas[J]. Plasma Science and Technology, 2018, 20(7): 74004-074004. DOI: 10.1088/2058-6272/aab5bc |
[6] | H R MIRZAEI, R AMROLLAHI. Design, simulation and construction of the Taban tokamak[J]. Plasma Science and Technology, 2018, 20(4): 45103-045103. DOI: 10.1088/2058-6272/aaa669 |
[7] | ZHU Yilun (朱逸伦), ZHAO Zhenling (赵朕领), TONG Li (仝丽), CHEN Dongxu (陈东旭), XIE Jinlin (谢锦林), LIU Wandong (刘万东). Optics System Design of Microwave Imaging Reflectometry for the EAST Tokamak[J]. Plasma Science and Technology, 2016, 18(4): 449-452. DOI: 10.1088/1009-0630/18/4/20 |
[8] | LI Gongshun (李恭顺), YANG Yao (杨曜), LIU Haiqing (刘海庆), JIE Yinxian (揭银先), ZOU Zhiyong (邹志勇), WANG Zhengxing (王正兴), ZENG Long (曾龙), WEI Xuechao (魏学朝), LI Weiming (李维明), LAN Ting (兰婷), ZHU Xiang (朱翔), LIU Yukai (刘煜锴), GAO Xiang (高翔). Bench Test of the Vibration Compensation Interferometer for EAST Tokamak[J]. Plasma Science and Technology, 2016, 18(2): 206-210. DOI: 10.1088/1009-0630/18/2/19 |
[9] | GAO Xiang (高翔), ZHANG Tao (张涛), HAN Xiang (韩翔), ZHANG Shoubiao (张寿彪), et al.. Observation of Pedestal Plasma Turbulence on EAST Tokamak[J]. Plasma Science and Technology, 2013, 15(8): 732-737. DOI: 10.1088/1009-0630/15/8/03 |
[10] | Naohiro KASUYA, Seiya NISHIMURA, Masatoshi YAGI, Kimitaka ITOH, Sanae-I ITOH. Heavy Ion Beam Probe Measurement in Turbulence Diagnostic Simulator[J]. Plasma Science and Technology, 2011, 13(3): 326-331. |
1. | Wang, S.Q., Zou, X.L., Duan, X.R. et al. Interaction between MTM and EGAM for energy and particle confinement improvements on HL-3 tokamak. Nuclear Fusion, 2025, 65(2): 026013. DOI:10.1088/1741-4326/ad9ba4 |