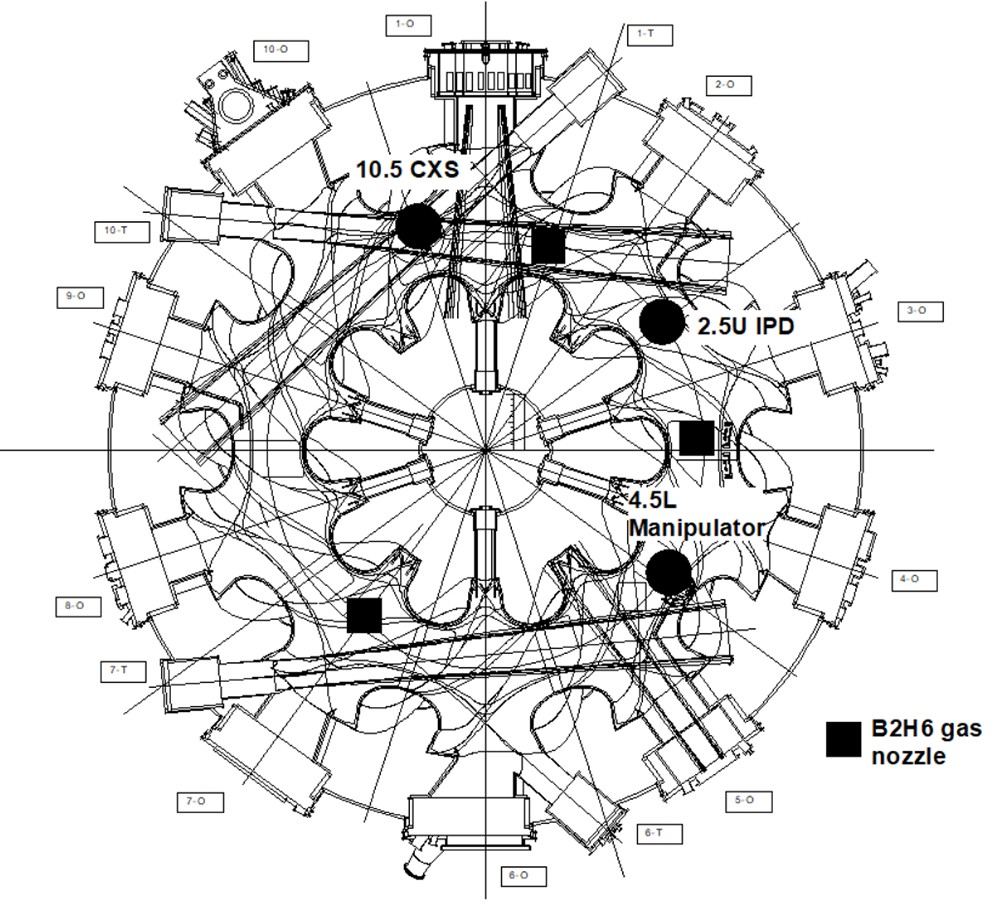
Citation: | Naoko ASHIKAWA, Robert LUNSFORD, Federico NESPOLI, Erik GILSON, Yaowei YU, Jiansheng HU, Shinichiro KADO. Coated boron layers by boronization and a real-time boron coating using an impurity powder dropper in the LHD[J]. Plasma Science and Technology, 2024, 26(8): 085103. DOI: 10.1088/2058-6272/ad495f |
In the Large Helical Device (LHD), diborane (B2H6) is used as a standard boron source for boronization, which is assisted by helium glow discharges. In 2019, a new Impurity Powder Dropper (IPD) system was installed and is under evaluation as a real-time wall conditioning technique. In the LHD, which is a large-sized heliotron device, an additional helium (He) glow discharge cleaning (GDC) after boronization was operated for a reduction in hydrogen recycling from the coated boron layers. This operational time of 3 h was determined by spectroscopic data during glow discharges. A flat hydrogen profile is obtained on the top surface of the coated boron on the specimen exposed to boronization. The results suggest a reduction in hydrogen at the top surface by He-GDC. Trapped oxygen in coated boron was obtained by boronization, and the coated boron, which has boron-oxide, on the first wall by B-IPD was also shown. Considering the difference in coating areas between B2H6 boronization and B-IPD operation, it would be most effective to use the IPD and B2H6 boronization coating together for optimized wall conditioning.
Boronization is one of the wall conditioning tools in fusion devices [1–4] since boron coatings are well known for their effect of reducing oxygen impurities due to easily formed chemical compounds such as boron-oxide. In the LHD, standard boron coatings are created by boronization, which uses diborane (B2H6) doped helium mixed gasses during glow discharges [5, 6]. Coated areas of boron by boronization are most importantly plasma-facing areas, including first walls and divertor targets. However, since B2H6 gas is toxic, caution is necessary during operation. In 2019, a new impurity powder dropper (IPD) system was installed [7, 8] to be tested as a real-time wall conditioning method. Using the IPD system, the amount and dropped timing of boron powders can be controlled. Due to the application method during active main discharges, the boron layer obtained using the IPD system is mainly considered for divertor targets.
In this paper, characterizations of coated boron layers by boronization and boron-IPD are analyzed. Especially since hydrogen isotope trapping by boron may contribute to wall recycling controls, analytical data of hydrogen isotope is shown. Hydrogen cycling control after boronization by helium glow discharges had been established using the spectroscopic data in the LHD. In addition, characterizations of three different boron coating methods, i.e. glow discharge boronization, ion cyclotron wall conditioning boronization and boron-IPD, are compared in this study.
In the LHD, boronization by means of B2H6 doped mixed helium (He) glow discharge has been operated. In early boronization in the LHD, the gas was introduced from one port at the 1.5 lower section. Then, the introduction of gas increased to three ports from 2002. In EAST, at the beginning of the project, boronization was carried out using C2B10H12 [9]. In addition, ion cyclotron wall conditioning (ICWC) [3, 4, 9] has been used for steady wall conditioning in EAST, and the method of adding C2B10H12 gas to the He-ICWC discharge was also implemented to apply a boron coat. Details of the operations are given in reference [10] and section 3.
Material manipulators are installed on both the LHD and EAST. In the LHD, in this work the material manipulator located in the 4.5 lower port was used, with the surface of specimens located at the first wall position. The location of the material manipulator is shown in figure 1. The material manipulator is separated by the vacuum gate valve between the plasma vacuum vessel and the manipulator, and up/down movement is controlled by the remote system. During the boronization operation, material exposure experiments can be performed by remote control. Even entry into the torus hall is not possible due to the toxicity of B2H6 gas.
In EAST, the manipulator was located at the midplane of a low-field side. A manipulator with a small head of about 5 cm was used, and the manipulators were installed when required for an experiment. The manipulator is also separated between the plasma vacuum vessel and the manipulator by the vacuum gate valve. However, the movement of the head with specimens to the plasma vacuum area was required to be done manually. Therefore, the head was installed at the first wall position before inserting B2H6 gas. When samples are removed from the head of manipulators, specimens are exposed to the atmosphere, and their top surface is oxidized in both manipulators in the LHD and EAST. The oxidizing effect of the atmosphere is known from previous studies [6].
The IPD system is installed at the 2.5 upper port in the LHD, as shown in figure 1. At present, two compositions, boron (B) and boron nitride (BN) powders can be used [7]. The powder dropping rate and an operational period are controlled by a piezo element and the typical flow rate is about 0.1–0.5 mg/s in this experiment. During the IPD experiment, the manipulator at the 4.5 lower port was used in the LHD, and details of the analytical data are shown in section 3.
Two kinds of surface analyses are used. Glow discharge optical emission spectroscopy (GD-OES) is used for depth profiles of compositions as qualitative analyses with argon (Ar) as the working gas and X-ray photoelectron spectroscopy (XPS) is utilized to obtain the depth profiles of atomic concentrations. XPS measures the chemical binding of compositions. GD-OES measures elements from hydrogen to high-Z materials. The GD-OES used in this study can measure hydrogen and deuterium separately. Target specimens and their history are listed in table 1. Further details of these specimens are discussed in section 3.
Name | Device | Base material | Boron coating | Additional plasma exposure | ||
Sample B1 | EAST | Si | C2B10H12 by ICWC | First wall position | − | − |
Sample B2 | LHD | SS316 | B2H6 by GDC | First wall position | − | − |
Sample B3 | LHD | SS316 | B power during H plasma | First wall position | − | − |
Sample B4 | LHD | SS316 | B2H6 by GDC | First wall position | About 20 D plasma discharges | First wall position |
First, based on the EAST and LHD experiments, table 2 shows the characteristics of the following three boron coatings. (1) C2B10H12 and He mixing gas during ICWC operation, (2) B2H6 gas and He mixing gas during glow discharge and (3) Boron powder droppings during the main plasma discharge.
Boronization: EAST | Boronization: LHD | Boron dropping: LHD | |
Working gas / conditioning plasma and the number of systems | Carborane (C2B10H12)+He / ICWC | Diborane (B2H6) + He / GDC | Boron powder (Diameter: 150 µm) |
1 (ICR antenna) | 2 (GDC anode) | Main plasma | |
1 s on / 2 s off | |||
Number of gas injection or dropping port | 1 | 3 | 1 |
Material of first wall / divertor | Mo / doped Graphite | SUS316 / Graphite | SUS316 / Graphite |
Wall temperature | Room temperature | Room temperature | Room temperature |
C : O : B (XPS) | 3 : 1 : 6 | 1 : 2 : 7 [6] | No data |
Operational characterizations | • Conditioning plasma, under confinement magnetic fields | • Conditioning plasma, no magnetic field | • Real-time boron coating under strong confinement magnetic fields |
Affected areas by ICWC are surfaces on the divertor and first wall, which have lines of sight from plasmas [11]. However, the area is limited compared to GDC | • Most widely coated boron is obtained on the divertor and first wall | • Coating area is the first wall (in this work) and might be divertor | |
• Injected B amounts: 10 g (in C2B10H12) | • Injected B amounts: 37 g (in B2H6) | • Dropped B powder: about 800 mg | |
Types of coated boron layer | • High carbon concentration is observed in the boron layer. Boron formed a binding with carbon in the coated layer. • The layer formed an effective boron- oxide and it was confirmed that there was trapped oxygen in the boron layer. | • Thick boron layer of 100–1000 nm was obtained per one boronization. • High purity of boron layer can be possible, and the pure boron affected additional oxygen trapping after boronization, such as main discharges. | • On the first wall, thin boron coating is obtained and hydrogen as the working gas of the main plasmas, and oxygen is trapped in the layer during the boron coating process. |
The most crucial point is the limitations and conditions regarding the operation of boronization methods. GDC and ICWC are applied during conditioning plasmas, while the IPD is operated during the main discharge. GDC is operated without magnetic fields, but ICWC and IPD are operated under magnetic fields. Thus, differences in base plasmas lead to different coated areas. Boron trapping efficiencies for hydrogen isotopes and oxygen by chemical binding in the boron layer are well known. However, real-time interactions and trappings between boron and other compositions are still under discussion. One of the candidate ideas is the interaction between boron powder and edge plasmas around the divertor regions based on spectroscopic observations [12].
The real-time interactions by boron can be operated by the IPD method only, and it is a unique technique that will even be used in future fusion devices. The results of the new analysis data are described after section 3.2.
Figure 2 shows the time evolution of hydrogen alpha (Hα) emission measured by visible spectroscopies, which was the charge exchange spectroscopy (CXS) [13] at the upper and lower ports of the 10.5 port in the LHD. Hα intensities over (a) 4 h and (b) 59 h as measured by the visible spectroscopies versus an elapsed time from 16:00, which is time = 0 min in figure 2, on January 12, 2002, are shown. During boronization, the flow of B2H6 gas began to decrease at 15 min, and the closing time of the B2H6 gas injection was 24 min. Arrows in figure 2 show the operational time of a helium (He) GDC, which was continuously operated during the whole boronization process.
The optical fibers connected to spectrometers were located at the 10.5 upper and lower ports, and an electric anode for the glow discharge was located at the midplane of the 10.5 section, in the plasma vacuum vessel. A B2H6 gas nozzle at the 1.5 lower port was used in the LHD experimental campaign. Two sets of the visible spectrometers were located close to the anode of glow discharge and the B2H6 gas injection nozzle. Intensities of Hα emission during glow discharges are uniform at different optical fiber lines on the cross-section of the viewport. Therefore, the intensities at the lower and upper spectrometers are approximately equivalent to each other.
A reduction in Hα intensity was observed continuously from 15 min until the end of He-GDC. The most significant reduction in Hα intensity was in the first 3 h. After 24 min, there was a reduction in hydrogen, as observed in Hα, which occurred due to the separation between B and H in B2H6 gas. However, a continuous decrease in hydrogen was still observed. The reason for this is thought to be that the hydrogen contained on the surface of the boron film coated on the plasma-facing wall was desorbed by the sputtering of the He glow discharge. The coated boron by boronization has the advantage of oxygen reduction and the disadvantage of higher recycling towards the next day’s plasma experiments, especially when plasma experiments with low electron density are required. The result in figure 2 shows the useful method of hydrogen reduction after boronization, which became the current operational scenario in the LHD, with additional He-GDC of 3 h after ending the B2H6 gas injection. Hydrogen recycling effects of coated boron on specimens are discussed in section 3.3.
Figure 3 shows depth profiles of compositions on specimens measured by GD-OES. Figures 3(a), (c) and (e) show the normalized intensities of carbon, calculated by dividing the deposited carbon intensities on the target specimens by the intensity obtained on the graphite standard sample, IG-430U. The normalized carbon amounts are over 1 at the top surface of the specimens. However, the top surface is influenced by air contamination, including carbide, and is not included in this discussion.
The boundary between the coating layer and the base specimens was determined as half intensities of materials for base specimens, which is iron (Fe) as the main component of stainless steel (SS) 316 or silicon (Si), and is shown by dashed lines. Figure 3(a) shows higher intensities of normalized carbon compared with the cases shown in figures 3(c) and (e), due to the carbon content of the working gas, C2B10H12, with the profile of the normalized carbon displaying higher intensity at the top surface of the coated B layer, and a decrease in signal deeper from the top surface.
In figure 3(b), which is the same specimen in the EAST experiment for figure 3(a), depth profiles of hydrogen and oxygen intensities are similar to carbon’s profile, such as reducing intensities towards deeper regions. In addition, carbon clearly remained in the boron layer of EAST. Higher hydrogen recycling after boronization, which used C2B10H12, was observed and it is a disadvantage of plasma operations in EAST. A profile of hydrogen with higher intensity at the top surface of the coated boron layer is considered to relate to higher hydrogen recycling, such as shown in figure 3(b).
In figure 3(d), a depth profile of hydrogen intensity can be seen from the top surface to an interface in a coated boron layer in the LHD. A gradual decrease was observed from t = 8 s to 0 s as the sputtered time, and it is considered that higher remaining hydrogen content at the top surface was removed by He-GDC after boronization. This surface analysis data is consistent with the trend of CX data for the 3 h of additional He-GDC shown in figure 1, which is effective in reducing hydrogen recycling.
Figure 3(f) shows a coated boron layer by boron powder dropping with a diameter of 150 μm, in hydrogen plasmas of IPD experiments on December 3 and 6 in 2019. An SS316 specimen was exposed to hydrogen plasmas using the material manipulator at the 4.5 lower port. The surfaces of the specimens were set at the first wall position during boron powder IPD experiments. The total amount of dropped boron powders is about 800 mg. This experiment uses strong magnetic fields to confine plasmas. Therefore, the coating on divertor targets was expected, but transport to the first wall was unknown. From the coated boron profile in figure 3(f), it is clearly shown that a boron coating from the IPD at the 2.5 section was deposited on the first wall at a different toroidal section, namely the 4.5 section, as shown in figure 1.
The changes in the depth profiles for hydrogen and oxygen are similar to each other in the coated boron layer. Therefore, it is considered that hydrogen and oxygen were trapped in boron layer formation. An advantage of B-IPD is that real-time interactions occur during the main plasma discharges. However, the extent of a longer lifetime of the coated layer is not clear. Nevertheless, it is not a serious issue as the limitation regarding the operational time of IPD is negligible compared with boronization.
Figure 4(a) shows a depth profile of atomic concentrations for a coated boron layer, in which the specimen, sample B1, exposed to ion cyclotron wall conditioning boronization with C2B10H12 of EAST, was measured by XPS. The coated thickness of the boron layer is about 45 nm, with the depth position determined by 50% of Si as a base specimen. The coated layer is comprised of 60% boron, 30% carbon and 10% oxygen. Carbon concentration in the layer is higher than that in the coated boron layer in the LHD [6], which is reasonable, considering the presence of carbon in C2B10H12.
Narrow profiles for boron, oxygen and carbon on sample B2 are analyzed, as shown in figures 4(b)–(d). The observed thickness of the EAST boron layer of about 34 nm as a hatched line, F4, is shown in figure 4(a), using XPS with Ar ion sputtering. In figures 4(b)–(d), the observed thickness of the LHD boron layer is about 14 nm from the top surface, measured by XPS. A depth profile for the specimen, sample B2, of the LHD is presented in reference [6]. In figure 4(b), the large amount of B–O binding in the LHD boron layer is shown. In figure 4(c), Highly Oriented Pyrolytic Graphite (HOPG) was measured to obtain a standard peak of C–C. The EAST boron layer shows a combination between C–C and C–B, and the LHD boron layer shows mainly a C–B peak, as shown in figure 4(c).
From fitting analyses for the area separation of B–B, B–C and B–O bindings, as shown in the peak positions of the boron narrow profile in figure 4(d), chemical compositions and concentrations for B–B (188.2 eV), B–C (189.5 eV) and B–O (192.0 eV) [14], in the B 1s peak of the EAST and LHD layers are analyzed, as shown in table 3. The EAST boron shows mainly boron-carbide (B–C) of 70%. The remaining amount of boron is observed as boron oxide (B–O), and pure boron was negligible in the layer of EAST. In the LHD, pure boron as B–B remains in the layer, and other bindings are B–O and B–C. When pure boron is facing plasmas after a sputtering by discharges, such as GDCs or main plasma discharges, the top surface of pure boron can trap impurities [6]. Hence, the concentration of pure boron remaining in the boron layers is important with respect to impurity reduction. It should also be noted that the top surface is changed by these sputtering processes.
B–B (%) | B–C (%) | B–O (%) | |
EAST (C2B10H12) | − | 70 | 30 |
LHD (B2H6) | 65 | 19 | 16 |
In figure 5, an SS316 specimen, namely sample B2, was exposed to boronization (B2H6) in the plasma vacuum vessel of the LHD using the material manipulator and subsequently removed. Later, one of the specimens after LHD boronization was exposed to deuterium main plasma discharges of about 20 shots at the first wall position using the material manipulator, and data of the specimen, namely sample B4, is also shown.
For both types of specimens with and without deuterium plasma exposure, oxygen intensities measured by GD-OES are compared, as shown in figure 5(a). The thickness of coated boron with deuterium main plasmas is thinner than that of coating without plasma exposure, indicating that the coated layer was eroded by the main plasmas. The intensities of oxygen in the coated boron layer with deuterium main plasmas are higher than those without plasma exposure. Therefore, the efficiency of oxygen trapping to the top surface of the coated boron is shown in figure 5(a).
Figure 5(b) shows intensities of compositions on the boron layer after boronization with plasma exposure on sample B4. An intensity of boron at the surface shows a reduction, and a peak of boron shows in the coated layer. The deuterium depth profile shows higher intensity at the top surface of the coated boron layer. An interaction with hydrogen isotopes on the coated boron layer has been reported to have the effect of shielding for hydrogen isotope permeation [15], and it is also considered that deuterium produced by main plasma discharges is retained only on the top surface of the coated layer. Therefore, comparing figures 5(a) and (b) clearly shows a difference in the trapping depth of oxygen and hydrogen after plasma exposure in the boron layers.
Thus, section 3 presents comprehensive characterizations of coated boron layers using surface analyses. Considering the difference in coating areas between B2H6 boronization and B-IPD operation, better operational scenarios may be obtained when these two techniques are used together.
Two boron coating methods, boronization as a wall conditioning and real-time boron coating by IPD, are operated in fusion devices, and their characteristics are summarized in this work.
In the LHD, a time evolution of reduction for hydrogen as a higher recycling source from the coated boron layer was observed. It was found that sufficient reduction of hydrogen was achieved after stopping B2H6 gas injection by carrying out a 3 h He-GDC. It has now become the standard operational scenario in the LHD.
Different types of coated boron layers, operated by C2B10H12 by He-ICWC, B2H6 by He-GDC, and B-IPD, are discussed. It was clarified that the top surface of the boron layer also contributes to oxygen trapping during the main discharges. A comparison of coated boron between C2B10H12 and B2H6 is shown, and the coated boron layer using C2B10H12 shows indications of chemical binding with carbon. Thus, the remaining high-purity boron layers are limited when compared with the boron layer by B2H6. It was revealed that the purer boron remains, the more oxygen impurities are trapped in the subsequent main discharges. Hence, the concentration of pure boron remaining in the boron layers is important for impurity reduction.
It was shown that a thin boron layer was coated on the first wall by B-IPD experiments, and material analysis revealed the effect of trapping oxygen and hydrogen at the top surface of the coated boron layer. Boron coating by B-IPD during the main discharge is considered to be more effective on the divertor region. However, this part was not evaluated in this experiment due to restrictions on using the manipulator. More details of real-time interactions between hydrogen isotopes and boron will be discussed in future works. Considering the difference in coating areas between B2H6 boronization and B-IPD operation, better operational scenarios may be obtained when these two techniques are used together.
Currently, only B2H6 can be produced in East Asia as a source of deuterated boron. JT-60SA plans to use B2D6 for their boronization. ITER started a discussion for additional wall conditioning, including boron coatings, relating to changing the first wall materials from beryllium to tungsten. Hence, it is important to understand the characteristics of coated boron walls.
This work is supported by NIFS budgets, KOBF031, ULFF004 and KUHR032. This research was partly supported by JSPS KAKENHI 18K04999, JSPS-CAS Bilateral Joint Research Projects, “Control of wall recycling on metallic plasma-facing materials in fusion reactor” 2019–2022, (No. GJHZ201984), the Chinese Academy of Sciences President’s International Fellowship Initiative Grant No. 2024VMB0003 in FY2023 and the U.S. Department Of Energy under Contract No. DE-AC02-09CH11466 with Princeton University.
Naoko Ashikawa gratefully acknowledges Dr Mamoru Shoji (NIFS) for operational support with the material manipulator in the LHD, Mr Junichi Yagyu (QST) for useful discussions about boronization, Dr Hyungho Lee (Korea Institute of Fusion Energy, Republic of Korea) for kind support in arranging an optical fiber procurement for IPD, and Dr Volker Rohde (Max Planck Institute for Plasma Physics, Germany) for valuable advice about hardware settings of IPD in a tokamak/helical hall.
[1] |
Winter J 1996 Plasma Phys. Control. Fusion 38 1503 doi: 10.1088/0741-3335/38/9/001
|
[2] |
Yagyu J et al 1997 J. Nucl. Mater. 241–243 579 doi: 10.1016/S0022-3115(96)00568-5
|
[3] |
Gu X M et al 2000 J. Plasma Fusion Res. Ser 3 320
|
[4] |
Li J G et al 2000 J. Plasma Fusion Res. Ser 3 40
|
[5] |
Nishimura K et al 2003 J. Plasma Fusion Res. 79 1216 doi: 10.1585/jspf.79.1216
|
[6] |
Ashikawa N et al 2007 J. Nucl. Mater. 363–365 1352 doi: 10.1016/j.jnucmat.2007.01.271
|
[7] |
Nespoli F et al 2020 Nucl. Mater. Energy 25 100842
|
[8] |
Lunsford R et al 2022 Nucl. Fusion 62 086021 doi: 10.1088/1741-4326/ac6ff5
|
[9] |
Wu J H et al 2011 J. Nucl. Mater. 415 S1046 doi: 10.1016/j.jnucmat.2010.10.089
|
[10] |
Hu J S et al 2009 Fusion Eng. Des. 84 2167 doi: 10.1016/j.fusengdes.2009.03.016
|
[11] |
Ashikawa N et al 2011 J. Plasma Fusion Res. 6 2402138 doi: 10.1585/pfr.6.2402138
|
[12] |
Kawate T et al 2022 Nucl. Fusion 62 126052 doi: 10.1088/1741-4326/ac9ac6
|
[13] |
Ida K et al 2001 Rev. Sci. Instrum. 71 2350
|
[14] |
Hu T et al 1998 Thin Solid Films 332 80 doi: 10.1016/S0040-6090(98)01019-0
|
[15] |
Tsuzuki K et al 1997 J. Nucl. Mater. 241–243 1055 doi: 10.1016/S0022-3115(96)00659-9
|
[1] | Zhe WANG, Zhen SUN, Guizhong ZUO, Kai WU, Yao HUANG, Wei XU, Ming HUANG, Zhitai ZHOU, Yanhong GUAN, Haotian QIU, Rajesh MAINGI, Jiansheng HU. Fuel recycling feedback control via real-time boron powder injection in EAST with full metal wall[J]. Plasma Science and Technology, 2024, 26(12): 125105. DOI: 10.1088/2058-6272/ad811f |
[2] | Liping DONG (董丽平), Yanmin DUAN (段艳敏), Kaiyun CHEN (陈开云), Xiuda YANG (杨秀达), Ling ZHANG (张凌), Feng XU (徐峰), Jingbo CHEN (陈竞博), Songtao MAO (毛松涛), Zhenwei WU (吴振伟), Liqun HU (胡立群). Influence of impurity seeding on the plasma radiation in the EAST tokamak[J]. Plasma Science and Technology, 2018, 20(6): 65102-065102. DOI: 10.1088/2058-6272/aab20c |
[3] | WANG Fuqiong(王福琼), CHEN Yiping(陈一平), HU Liqun(胡立群). DIVIMP Modeling of Impurity Transport in EAST[J]. Plasma Science and Technology, 2014, 16(7): 642-649. DOI: 10.1088/1009-0630/16/7/03 |
[4] | MING Tingfeng (明廷凤), Satoshi OHDACHI, Yasuhiro SUZUKI, LHD Experiment Group. Estimate of the Deposition Profile of Carbon Pellets Using a High-Speed VUV Imaging System in the LHD[J]. Plasma Science and Technology, 2013, 15(12): 1178-1184. DOI: 10.1088/1009-0630/15/12/03 |
[5] | Kenji SAITO, Ryuhei KUMAZAWA, Tetsuo SEKI, Hiroshi KASAHARA, Goro NOMURA, et al. Measurement of Ion Cyclotron Emissions by Using High-Frequency Magnetic Probes in the LHD[J]. Plasma Science and Technology, 2013, 15(3): 209-212. DOI: 10.1088/1009-0630/15/3/03 |
[6] | WANG Erhui (王二辉), S. MORITA, M. GOTO, DONG Chunfeng (董春凤). Observation of the Two-Dimensional Distribution of Impurity Line Emissions Using a Space-Resolved EUV Spectrometer in LHD[J]. Plasma Science and Technology, 2013, 15(2): 106-109. DOI: 10.1088/1009-0630/15/2/05 |
[7] | Hiroe IGAMI, Hiroshi IDEI, Shin KUBO, Yasuo YOSHIMURA., Takashi SHIMOZUMA, Hiromi TAKAHASHI. Measurement of the electron Bernstein wave emission with one of the power transmission lines for ECH in LHD[J]. Plasma Science and Technology, 2011, 13(4): 405-409. |
[8] | DONG Chunfeng, Shigeru MORITA, Motoshi GOTO, Masahiro KOBAYASHI. Study on Radial Position of Impurity Ions in Core and Edge Plasma of LHD Using Space-Resolved EUV Spectrometer[J]. Plasma Science and Technology, 2011, 13(2): 140-144. |
[9] | ZUO Guizhong, HU Jiansheng, LI Jiangang, LUO Nanchang, HU Liqun, FU Jia, CHEN Kaiyun, TI Ang, ZHANG Lili. Primary Results of Lithium Coating for the Improvement of Plasma Performance in EAST[J]. Plasma Science and Technology, 2010, 12(6): 646-650. |
[10] | WANG Junyi (王君一), CHEN Yiping(陈一平). Study of Carbon Impurity Transport at SOL in EAST[J]. Plasma Science and Technology, 2010, 12(5): 535-539. |
Name | Device | Base material | Boron coating | Additional plasma exposure | ||
Sample B1 | EAST | Si | C2B10H12 by ICWC | First wall position | − | − |
Sample B2 | LHD | SS316 | B2H6 by GDC | First wall position | − | − |
Sample B3 | LHD | SS316 | B power during H plasma | First wall position | − | − |
Sample B4 | LHD | SS316 | B2H6 by GDC | First wall position | About 20 D plasma discharges | First wall position |
Boronization: EAST | Boronization: LHD | Boron dropping: LHD | |
Working gas / conditioning plasma and the number of systems | Carborane (C2B10H12)+He / ICWC | Diborane (B2H6) + He / GDC | Boron powder (Diameter: 150 µm) |
1 (ICR antenna) | 2 (GDC anode) | Main plasma | |
1 s on / 2 s off | |||
Number of gas injection or dropping port | 1 | 3 | 1 |
Material of first wall / divertor | Mo / doped Graphite | SUS316 / Graphite | SUS316 / Graphite |
Wall temperature | Room temperature | Room temperature | Room temperature |
C : O : B (XPS) | 3 : 1 : 6 | 1 : 2 : 7 [6] | No data |
Operational characterizations | • Conditioning plasma, under confinement magnetic fields | • Conditioning plasma, no magnetic field | • Real-time boron coating under strong confinement magnetic fields |
Affected areas by ICWC are surfaces on the divertor and first wall, which have lines of sight from plasmas [11]. However, the area is limited compared to GDC | • Most widely coated boron is obtained on the divertor and first wall | • Coating area is the first wall (in this work) and might be divertor | |
• Injected B amounts: 10 g (in C2B10H12) | • Injected B amounts: 37 g (in B2H6) | • Dropped B powder: about 800 mg | |
Types of coated boron layer | • High carbon concentration is observed in the boron layer. Boron formed a binding with carbon in the coated layer. • The layer formed an effective boron- oxide and it was confirmed that there was trapped oxygen in the boron layer. | • Thick boron layer of 100–1000 nm was obtained per one boronization. • High purity of boron layer can be possible, and the pure boron affected additional oxygen trapping after boronization, such as main discharges. | • On the first wall, thin boron coating is obtained and hydrogen as the working gas of the main plasmas, and oxygen is trapped in the layer during the boron coating process. |
B–B (%) | B–C (%) | B–O (%) | |
EAST (C2B10H12) | − | 70 | 30 |
LHD (B2H6) | 65 | 19 | 16 |