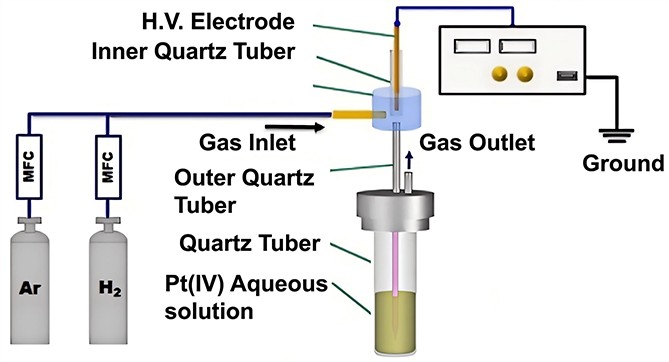
Citation: | Yuxuan HUANG, Ming ZHANG, Yong YANG, Fangwei LYU, Xiaopeng YI, Chaofan LYU, Yisong ZHANG, Bo RAO. Design and experimental study of a field-reversed configuration plasma thruster prototype[J]. Plasma Science and Technology, 2025, 27(3): 035507. DOI: 10.1088/2058-6272/ada376 |
The field-reversed configuration (FRC) plasma thruster driven by rotating magnetic field (RMF), abbreviated as the RMF-FRC thruster, is a new type of electric propulsion technology that is expected to accelerate the deep space exploration. An experimental prototype, including diagnostic devices, was designed and constructed based on the principles of the RMF-FRC thruster, with an RMF frequency of 210 kHz and a maximum peak current of 2 kA. Under the rated operating conditions, the initial plasma density was measured to be 5 × 1017 m−3, and increased to 2.2 × 1019 m−3 after the action of RMF. The coupling efficiency of RMF was about 53%, and the plasma current reached 1.9 kA. The axial magnetic field changed in reverse by 155 Gauss, successfully reversing the bias magnetic field of 60 Gauss, which verifies the formation of FRC plasma. After optimization research, it was found that when the bias magnetic field is 100 Gauss, the axial magnetic field reverse variation caused by FRC is the highest at 164 Gauss. The experimental results are discussed and strategies are proposed to improve the performance of the prototype.
Nanomaterials exhibit significant surface effects, interface effects, and small size effects in natural science due to their small particle size and large specific surface area [1‒4]. In particular, noble metal nanomaterials possess both unfilled d-orbital valence layer and dual properties of transition metals and nanomaterials [5‒8]. The advantages of platinum (Pt) are particularly prominent. Platinum nanomaterials (PtNPs), which are widely regarded as the first choice for catalysts and are hot research objects in the field of catalysis, can provide diverse surface electronic states and surface atomic coordination vacancies, and have excellent catalytic activity [9‒10]. Notably, PtNPs, due to their excellent biocompatibility and antibacterial properties, have numerous applications in biomedical field [5, 11‒14], such as tumor treatment, nano-diagnosis, treatment of oxidative stress-related diseases, antibacterial agents, and skin preparations. However, chemical reductants commonly used in liquid-phase method exhibit long reaction time, requiring high-temperature reactions and further treatment with auxiliaries. As the primary crystal surface of common units such as spheres, cubes, octahedrons, truncated cubes, and truncated octahedrons, the crystal face (111) tends to attract target molecules due to its lowest free energy, rather than other high-energy crystal faces [15‒17]. The preferential adsorption behavior contributes to increasing the coverage of target molecules on the crystal surface, which may further affect and regulate the overall properties of the material. By optimizing the exposure degree of the crystal face (111), the activity and selectivity of catalytic reactions can be effectively regulated. Frequently, to obtain nanoparticles that grow with (111) crystal plane orientation, surfactants such as polyvinylpyrrolidone (PVP) and sodium citrate are required [18‒20]. Surfactants can easily bind with the inert protective layer formed on the metal surface, which is difficult to remove. This can lead to background interference signals that affect analytical detection and impair the activity of nanoparticles. The solvent chemical reduction method cannot achieve efficient preparation in terms of reaction rate, product quality, and subsequent treatment. Therefore, it is particularly important to develop an appropriate method to prepare the PtNPs that primarily grow on the platinum (111) crystal plane.
Considering the constraints, scientists have been relentlessly exploring novel and efficient synthetic pathways aimed at precisely controlling the shape, size, and orientation of nanoparticles [21‒23]. Under mild operational conditions precious metals can be efficiently and selectively reduced to synthesize nanoparticles with the (111) surface predominantly serving as the primary crystal plane, thereby facilitating precise material design [24‒26]. Plasma, as a novel green approach, has been proposed to act as an efficient reducing agent compared to the other chemical agent which would introduce the problems of environment pollution. In the author’s previous work [27‒29], Pt-based catalysts with graphene as the carrier, which exhibit good dispersion and a narrow particle size range, were first prepared using low-pressure radio frequency discharge plasma. Subsequently, an atmospheric pressure plasma jet (APPJ) was generated and employed for the gas-solid phase reduction of graphene oxide and the Pt-based catalysts with reduced graphene oxide as the carrier. It was discovered that APPJ possesses excellent reduction effects. This study further explores the potential of low-temperature plasma technology in preparation of pure Pt nanomaterials. In this work, authors attempted to reduce Pt(IV) ions in the liquid phase using APPJ and prepared unsupported PtNPs with good dispersion and uniform particle size distribution.
Chloropplinate hexahydrate (H2PtCl6·6H2O) was used as the precursor with a concentration of 0.04 g·L−1 Pt(IV) aqueous solution. The details of the dielectric barrier discharge (DBD) equipment have been described in reference [29]. As shown in figure 1, the plasma jet flows directly into the Pt(IV) ion aqueous solution contained in a quartz reactor chamber through a quartz tube. 60.0 mL of the above-described Pt(IV) ionic solution was transferred into the quartz reactor. The APPJ was generated in a mixture of Ar (2.5 L/min) and H2 (0‒40 mL/min) with applied voltage about 7.0 kV. The plasma generated with the mixture is in direct contact with the solution in the reactor chamber. The reaction time was set as 3 min and 5 min, respectively. In situ diagnosis was performed using an OES emission spectrometer (Avaspec 2048, The Netherlands) with a multi-channel fast camera (CCD). The scanning wavelength ranged from 200 to 1100 nm with an accuracy of 0.1 nm. Emission spectra were collected and stored by a computer.
Figure 2 depicts the plasma emission spectrum, encompassing a wavelength range of 480–550 nm, under conditions of applied voltage 7 kV, a steady flow rate of Ar gas 3 L·min−1, and the flow rate of H2 40 mL/min. As shown in figure 2, the APPJ contains a large number of particles with excited states or metastable states, which can provide high-energy active electrons to noble metal ions in the reaction and directly reduce the noble metal ions avoiding harsh reaction conditions such as high-temperature hydrogenation.
Referring to reaction (1), the discharge gas is excited by high-energy electrons and forms many excited Ar atoms and electrons. Most of the excitation states exist in the form of Ar I atomic line (495.67 nm), which is a characteristic spectral line resulting from the transition of outer electron energy level in Ar atomic nucleus, with excitation potential of 11.67 eV. Ar II atomic lines, which have higher energy than Ar I atomic lines, are the characteristic spectral lines produced by the transition of outer energy level of Ar ion [30].
Ar+e∗→Ar·+e | (1) |
The presence of Ar promotes the Penning dissociation effect. It significantly improves the collision frequency between neutral gas molecules and free electrons. As a result, it greatly promotes the dissociation process of H2 shown in reactions (2) and (3). As can be seen from figure 2, the characteristic spectral line of Hβ (486.13 nm) appears with an excitation potential of 2.556 eV after adding H2. Since the ionization energy of ground-state hydrogen atoms is 13.60 eV, high-energy Hβ particles are produced in the plasma jet.
Ar·+H2→Ar+2H· | (2) |
H2+e∗→2H·+e | (3) |
Figure 3 shows the XPS of PtNPs, which were synthesized via the APPJ. The characteristic peaks of electron binding energy are evident at 74.35 eV (Pt 4f5/2) and 71.00 eV (Pt 4f7/2), with a peak spacing of 3.35 eV. In the context of the spin splitting spectrum, the spacing between the double peaks serves as a crucial indicator for determining the chemical state of the element. The Pt atoms within the PtNPs exist solely in the form of zero-valent Pt atoms, suggesting that Pt(IV) has been effectively reduced to Pt. Generally, chloroplatinic acid is a stable complex, and the coordination bond enables it to have higher thermal stability and chemical stability. It is imperative to initially disrupt the Pt–Cl coordination bond to obtain PtNPs.
The process of chemically reducing Pt(IV), which essentially involves the electron transfer between the reducing agent and Pt(IV), often necessitates a prolonged reaction time due to the nature of its reaction mechanism. Additionally, surfactants are frequently utilized to aid in controlling crystal formation and enhancing reaction efficiency. The process, typically consisting of the following two steps, has standard electrode potentials of 0.68 V and 0.73 V respectively.
[PtCl6]2−+2e−=[PtCl4]2−+2Cl−, ϕ(Pt4+/Pt2+)⊖=0.68V | (4) |
[PtCl4]2−+2e−=Pt0+4Cl−, ϕ(Pt2+/Pt0)⊖=0.73V | (5) |
Firstly, the high-energy electrons generated by the plasma can be utilized directly as reductants [31]. When the plasma jet is injected into the solution containing Pt(IV) ion precursors, the electrons generated by the plasma jet penetrate the solution significantly, leading to the formation of solvated electrons (eaq). Due to electrostatic attraction, eaq is drawn towards the Pt(IV) ions. On the surface of metal particles, electron layers gradually form, effectively limiting the size of nanoparticles because of the electrostatic repulsion exerted by these electron layers. Subsequently, solvated electrons reduce Pt(IV) ions to elemental Pt through a specific reaction (6).
Pt4++4eaq→Pt0 | (6) |
Secondly, the APPJ contains a large number of free H atoms and Ar atoms. As the metastable excited state energy of argon is higher than the ionization energy of H atoms, the Penning effect will occur, which is conducive to the ionization reaction (7) of Hβ and thus generates electrons (e) and hydrogen ions (H+). Subsequently, Pt(IV) ions can be reduced to elemental Pt through specific chemical reactions (8) and (9).
Ar·+H·→Ar+H++e− | (7) |
Pt4++4H·→Pt0+4H+ | (8) |
Pt4++2Ar·+2H·→Pt0+2Ar+2H+ | (9) |
The commonly used chemical reduction method mentioned earlier requires the addition of a certain amount of reducing agent, typically hydrazine hydrate or NaBH4. In this type of reaction, the reducing agent supplies electrons to reduce Pt(IV) ions into Pt atoms, which then form PtNPs. The concentration of the reducing agent and the pH value in this method both influence the charge state of ions in the solution, thereby directly affecting the reaction rate and the degree of reduction. The addition of reducing agents and pH regulators alters the ionic environment of the solution where the reaction occurs, making it more complex. The type and concentration of ions in this environment can have a certain influence on the nucleation and growth processes of nanoparticles. Therefore, to obtain Pt (111) facets with lower surface energy through the chemical reduction method, it is necessary to precisely control the concentration, pH, and temperature, or to add specific surfactants and adjust the ion concentration to achieve fine control over the shape and size of nanoparticles. The plasma approach, on the other hand, leverages the energy from plasma to excite and ionize atoms, which can lead to the formation of nanoparticles with a more uniform size distribution and a higher degree of control over the crystalline plane orientation. This is due to the lower process temperatures and the absence of additional chemical reagents, which can introduce impurities or lead to unwanted side reactions.
Therefore, the presence of these high-energy active particles, particularly solvated electrons, within the plasma jet prompts a swift and thorough reaction with the Pt(IV) ion at the coordination center. This reaction effectively reduces the Pt(IV) to Pt(0) atoms, resulting in the disappearance of the coordination structure and the dissociation of the coordination Cl−. The ion environment of the reaction system treated by plasma is relatively simple and has a relatively stable rule, which is more conducive to obtaining Pt (111) crystal plane with lower surface energy.
As shown in figure 4, within the diffraction angle range of 35°‒85°, the diffraction angles of the characteristic peaks in the X-ray diffraction (XRD) spectrum of PtNPs are 39.73°, 46.2°, 67.4°, and 81.2°, respectively. Compared with the Pt XRD standard card JCPDS-040802, the crystal planes of (111), (200), (220), and (311) are aligned, revealing a face-centered cubic structure (fcc) for the crystal structure of PtNPs.
As can be seen from the high-resolution transmission electron microscopy (TEM) in figure 5, the exposed crystal plane of granular Pt nanocrystals is flat (111), and the lattice spacing of PtNPs is about 0.226 nm, which is consistent with the lattice spacing of Pt (111) crystal plane. The presence of Cl− in solution significantly contributes to this result, as halogen ions exert a promoting effect on the growth of the (111) crystal plane. The Cl− ions alter the charge distribution and energy state of the crystal surface, ultimately influencing both the growth rate and direction of the crystal. It can be adsorbed on the surface of the crystal to reduce the surface energy, making it easier for the crystal to grow on the (111) crystal plane, and ultimately exposing the crystal plane of nanocrystals. Therefore, the PtNPs crystal prepared is mainly composed of a flat Pt (111) crystal plane with small particle size and local defects on the surface.
The growth of metal nanoparticles in solutions usually involves two key stages [32‒33]. First, metal precursors undergo reduction reactions in the liquid phase to form solid-phase crystal nuclei. Subsequently, these solid-phase crystal nuclei gradually increase in size and eventually develop into nanocrystals. According to crystal growth theory, as the concentration of zero-valent metal atoms in the solution continues to rise and reaches saturation, these atoms precipitate from the liquid phase to form solid-phase crystal nuclei. The newly generated Pt(0) tends to attach and grow on the existing solid-phase crystal nuclei, leading to a gradual increase in the size of the crystal nuclei until a dynamic equilibrium is reached between the metal atoms on the surface of the nanocrystals and the zero-valent metal atoms in the solution. If a large number of crystal nuclei can be rapidly generated in the solution and the atoms can reach a supersaturated state in a short time, then it is expected to obtain nanoparticles with finer particle sizes.
In the presence of the carrier, e.g. reduced graphene oxide, PtNPs were uniformly dispersed with a particle size distribution ranging from 2.0 to 5.0 nm. The carrier had many active sites on the surface, including –OH, –COOH, –CH(O)CH–, and surface defect sites. The existence of these active sites could anchor Pt(IV) ions on the graphene carrier in the form of coordination bonds and chemical adsorption, allowing reduction, nucleation, and growth to occur on the carrier. Therefore, there was almost no agglomeration of metal particles, whereas graphene carriers tended to agglomerate. In this work, the APPJ reduction is extremely rapid without any surfactant and carrier. Upon introducing APPJ, Pt(IV) ions undergo rapid reduction to Pt(0) atoms in the solution, expeditiously forming Pt nanocrystalline nuclei. Experimental findings reveal that PtNPs treated with the APPJ exhibit a spherical-like shape and a concentrated particle size distribution. Additionally, it is evident that the treatment time significantly affects the particle size of PtNPs [25]. Nanoparticles ranging from 1.0 to 3.6 nm can be produced within 3 min of treatment time, with an average size of 2.2 nm, due to rapid supersaturation of atoms. At this point, the solution forms a stable sol system, and the prepared PtNPs can be redispersed in the ethanol solution. As figures 5(d) and (e) demonstrate, small particles are gradually brought together to form vellus clusters of varying sizes which are attributed to the fact that a small quantity of free Pt(0) continues to grow on the existing solid phase crystal nucleus with the extension of the treatment time, resulting in a gradual increase in the crystal nucleus size.
The system treated with APPJ exhibits sol characteristics. Zeta potential analysis was used to investigate the surface charge and stability of PtNPs. The results showed that the PtNPs sol system treated by APPJ had negative zeta potential, indicating that the colloidal particles carried negative charges, and the treatment time had a significant impact on the charge characteristics and stability of the sol system. As shown in figure 6(a), as the reaction proceeds the absolute value of the zeta potential gradually decreases. The zeta potential ζ of the PtNPs sol system is −56.0 mV, −31.6 mV, −20.3 mV, and −12.5 mV for treatment time of 2 min, 3 min, 4 min, and 5 min, respectively. The absolute value of the ζ diminished due to several factors. During the initial phase of nanoparticles formation, the surfaces of these particles possess numerous charges due to their size and low concentration, resulting in a substantial electrostatic repulsion among them. Therefore, the sol has high stability and a relatively high ζ potential. In the process of PtNPs formation, H+ ions in the sol may be adsorbed on the particle surface, thus neutralizing the surface charges. The charge neutralization can lead to a decrease in the absolute value of ζ potential. As shown in figure 6(a), the pH value continuously dropped from 2.85 to 1.62 because H• enters and converts to H+ as the reaction proceeds, increasing the concentration of H+ and causing a slight pH decrease.
The experimental phenomena revealed that aggregation and sedimentation became evident with the increase of processing time, indicating a gradual instability in the sol system. Prior experimental findings also demonstrated that the PtNPs underwent continual modifications in both size and morphology throughout the reaction process. Specifically, at the 5 min mark, the nanoparticles enlarged, leading to a reduction in their specific surface area. Consequently, the decrease in surface area may have diminished the surface charge density, also resulting in a decrease in the absolute value of the ζ potential, thus explaining the aforementioned phenomenon. The formed PtNPs are still difficult to disperse uniformly in ethanol.
The temperature rise will accelerate the formation of nanoparticles and the change of surface charge as the temperature of the device cannot be dissipated in time. A longer reaction time may lead to more charge neutralization and particle agglomeration. In addition, the continuous influx of high-energy particles attached to the surface of nanoparticles affects their surface properties, increases the probability of collisions between particles, and also causes agglomeration. According to the characterization and discussion of the previous text, the formation mechanism of PtNPs prepared by APPJ in liquid phase with high efficiency is further proposed. The schematic diagram can be seen in figure 7. High-energy active particles in APPJ undergo reduction reactions with Pt(IV) ions in the liquid phase, breaking Pt–Cl coordination bonds. Pt(IV) rapidly gain electrons and are reduced to form a large amount of Pt(0), which aggregates and grows directionally to form nanoparticles that serve as colloidal cores in this system. Successfully reduced through the APPJ reduction reaction, Pt(IV) ions transformed into PtNPs, serving as colloidal cores. Consequently, the concentration of Pt(IV) ions in the solution declined precipitously due to their extensive consumption during the reaction, whereas the concentration of Cl− ions rose comparatively. Cl− ions possess weaker hydration capacity, implying that they are more prone to detach from water molecules and participate in other chemical reactions or adsorption behaviors. In this case, Cl− ions are adsorbed on the surface of colloidal particles as locating ions through non-selective adsorption. This adsorption is non-specific, meaning that Cl− ions do not adsorb specifically to a particular location on the colloidal particles, but are randomly distributed on the surface of the colloidal particles. As Cl− ions are adsorbed, negative colloidal particles are gradually formed. These colloidal particles carry negative charges due to the adsorption of Cl− ions, thus forming a stable negative colloidal system. H+ ions do not directly adsorb on the surface of the colloidal core, but exist as counter-located ions, forming an adsorption layer and a diffusion layer. The structural formula of the micelle can be expressed as [(Pt)m·nCl−·(n−x)H+]·x H+. The adsorption layer and diffusion layer formed by H+ form an ionic atmosphere, which can prevent aggregation between particles and maintain the stability of the sol system. A stable sol system can be formed when the treatment time is 3 min. However, as the treatment time reaches 5 min, the sol system begins to exhibit instability because the attractive forces between colloidal particles gradually dominates, leading to particle aggregation. By reasonably controlling the adsorption process of Cl− ions and H+ ions, stable sol systems can be prepared and play crucial roles. It is necessary to further explore the effects of different ions and stabilizers on the stability of sol systems to achieve broader applications.
In conclusion, the study has successfully demonstrated the potential of the synthesis of PtNPs by APPJ in liquid phase. Through OES and XPS tests, it was found that the plasma jet contains a large number of high-energy active particles, which generate solvated electrons in the liquid phase. These electrons promote the rapid reduction of Pt(IV) ions in the solution to Pt(0) atoms, forming Pt nanocrystalline nuclei that gradually grow into nanoparticles. After 3 min of treatment, the PtNPs exhibit excellent dispersion and a narrow particle size distribution of 1.8‒2.8 nm, presenting in a colloidal state. However, when the treatment time is extended to 5 min, the particles are likely to grow and may undergo aggregation and sedimentation due to changes in ion concentration, pH levels, and temperature within the solution. The results of XRD and TEM confirm that PtNPs mainly exist in the (111) crystal plane. The absolute value of the zeta potential decreases from 56.0 mV to 12.5 mV, verifying the effect of treatment time on the stability of the colloidal system. The APPJ reduction process is proved to be efficient and rapid, requiring no surfactants, providing a novel approach for the efficient and controllable preparation of PtNPs.
This work was supported by National Natural Science Foundation of China (NSFC) (Nos. 62201217 and 51821005).
[1] |
Polzin K et al 2020 Aerospace 7 105 doi: 10.3390/aerospace7080105
|
[2] |
Sun X F et al 2018 J. Rocket Propuls. 44 44 (in Chinese) doi: 10.3969/j.issn.1672-9374.2018.01.007
|
[3] |
Brown N P and Walker M L R 2020 Appl. Sci. 10 3775 doi: 10.3390/app10113775
|
[4] |
Furukawa T, Kuwahara D and Shinohara S 2021 Phys. Plasmas 28 073507 doi: 10.1063/5.0035383
|
[5] |
Furukawa T et al 2017 AIP Adv. 7 115204 doi: 10.1063/1.4998248
|
[6] |
Weber T E, Slough J T and Kirtley D 2012 Rev. Sci. Instrum. 83 113509 doi: 10.1063/1.4759000
|
[7] |
Bathgate S N, Bilek M M M and Mckenzie D R 2017 Plasma Sci. Technol. 19 083001 doi: 10.1088/2058-6272/aa71fe
|
[8] |
Thonemann P C, Cowhig W T and Davenport P A 1952 Nature 169 34 doi: 10.1038/169034a0
|
[9] |
Slough J T and Miller K E 2000 Phys. Plasmas 7 1945 doi: 10.1063/1.874019
|
[10] |
Steinhauer L C 2011 Phys. Plasmas 18 070501 doi: 10.1063/1.3613680
|
[11] |
Glasser A H and Cohen S A 2022 Rev. Sci. Instrum. 93 083506 doi: 10.1063/5.0101665
|
[12] |
Velas K M and Milroy R D 2014 Phys. Plasmas 21 012502 doi: 10.1063/1.4861131
|
[13] |
Sercel C L, Gill T M and Jorns B A 2023 Plasma Sources Sci. Technol. 32 105017 doi: 10.1088/1361-6595/acfd5a
|
[14] |
Weber T 2010 The electrodeless Lorentz force thruster experiment PhD Thesis University of Washington, Seattle, USA
|
[15] |
Gill T M, Sercel C L and Jorns B A 2024 Plasma Sources Sci. Technol. 33 015006 doi: 10.1088/1361-6595/ad107a
|
[16] |
Furukawa T et al 2018 Rev. Sci. Instrum. 89 043505 doi: 10.1063/1.5013214
|
[17] |
Furukawa T et al 2017 Phys. Plasmas 24 043505 doi: 10.1063/1.4979677
|
[18] |
Liu X X et al 2022 IEEE Trans. Plasma Sci. 50 2138 doi: 10.1109/TPS.2022.3175526
|
[19] |
Jones I R and Hugrass W N 1981 J. Plasma Phys. 26 441 doi: 10.1017/S0022377800010837
|
[20] |
Slough J T and Miller K E 2000 Phys. Rev. Lett. 85 1444 doi: 10.1103/PhysRevLett.85.1444
|
[21] |
Milroy R D 1999 Phys. Plasmas 6 2771 doi: 10.1063/1.873234
|
[22] |
Milroy R D 2000 Phys. Plasmas 7 4135 doi: 10.1063/1.1290279
|
[1] | Xianhai PANG (庞先海), Zixi LIU (刘紫熹), Shixin XIU (修士新), Dingyu FENG (冯顶瑜). Arc characteristics during the instability stage on transverse magnetic field contacts[J]. Plasma Science and Technology, 2018, 20(9): 95505-095505. DOI: 10.1088/2058-6272/aac50a |
[2] | XU Yan (徐艳), ZHANG Xiaoqing (张晓晴), YANG Chunhui (杨春辉), ZHANG Yanping (张燕平), YIN Yongxiang (印永祥). Recent Development of CO2 Reforming of CH4 by “Arc” Plasma[J]. Plasma Science and Technology, 2016, 18(10): 1012-1019. DOI: 10.1088/1009-0630/18/10/08 |
[3] | JU Xingbao (琚兴宝), SUN Haishun (孙海顺), YANG Zhuo (杨倬), ZHANG Junmin (张俊民). Investigation on the Arc Ignition Characteristics and Energy Absorption of Liquid Metal Current Limiter Based on Self-Pinch Effect[J]. Plasma Science and Technology, 2016, 18(5): 531-537. DOI: 10.1088/1009-0630/18/5/15 |
[4] | ZHONG Jianying (钟建英), GUO Yujing (郭煜敬), ZHANG Hao (张豪). Research of Arc Chamber Optimization Techniques Based on Flow Field and Arc Joint Simulation[J]. Plasma Science and Technology, 2016, 18(3): 319-324. DOI: 10.1088/1009-0630/18/3/17 |
[5] | Seon-Geun OH, Young-Jun LEE, Jae-Hong JEON, Jong-Hyeon SEO, Hee-Hwan CHOE. The Spatial Effects of Antenna Configuration in a Large Area Inductively Coupled Plasma System for Flat Panel Displays[J]. Plasma Science and Technology, 2014, 16(8): 758-766. DOI: 10.1088/1009-0630/16/8/06 |
[6] | Jee-Hun KO, Sooseok CHOI, Hyun-Woo PARK, Dong-Wha PARK. Decomposition of Nitrogen Trifluoride Using Low Power Arc Plasma[J]. Plasma Science and Technology, 2013, 15(9): 923-927. DOI: 10.1088/1009-0630/15/9/17 |
[7] | CHEN Wenguang (陈文光), RAO Jun (饶军), LI Bo (李波), LEI Guangjiu (雷光玖), CAO Jianyong (曹建勇), WANG Mingwei (王明伟), KANG Zihua (康自华), FENG Kun (冯鲲), HL-A NBI Group. Technical Design of Arc-Discharge and Deceleration Power Supply for MW Level NBI System on HL-2A Tokamak[J]. Plasma Science and Technology, 2012, 14(10): 936-940. DOI: 10.1088/1009-0630/14/10/15 |
[8] | LI Hui (李辉), XIE Mingfeng(谢铭丰). Measurement of Plasma Parameters of Gliding Arc Driven by the Transverse Magnetic Field[J]. Plasma Science and Technology, 2012, 14(8): 712-715. DOI: 10.1088/1009-0630/14/8/06 |
[9] | BAI Bing (白冰), ZHA Jun (査俊), ZHANG Xiaoning (张晓宁), WANG Cheng (王城), XIA Weidong (夏维东). Simulation of Magnetically Dispersed Arc Plasma[J]. Plasma Science and Technology, 2012, 14(2): 118-121. DOI: 10.1088/1009-0630/14/2/07 |
[10] | MU Zongxin (牟宗信), WANG Chun (王春), MU Xiaodong (牟晓东), JIA Li (贾莉), LIU Shengguang (刘升光), DONG Chuang(董闯). Experimental Study of the Effect of Applied Magnetic Field on Plasma Properties of Unbalanced Magnetron Sputtering[J]. Plasma Science and Technology, 2010, 12(5): 571-576. |