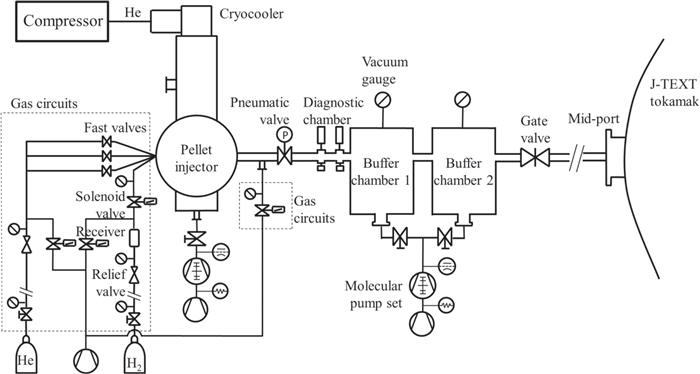
Citation: | Dingchen LI, Chuan LI, Jiawei LI, Wendi YANG, Menghan XIAO, Ming ZHANG, Yong YANG, Kexun YU. Efficient direction-independent fog harvesting using a corona discharge device with a multi-electrode structure[J]. Plasma Science and Technology, 2022, 24(9): 095502. DOI: 10.1088/2058-6272/ac6be4 |
Efficient collection of water from fog can effectively alleviate the problem of water shortages in foggy but water-scarce areas, such as deserts, islands and so on. Unlike inefficient fog meshes, corona discharge can charge water droplets and further enhance the water-collecting effect. This study proposes a novel multi-electrode collecting structure that can achieve efficient and direction-independent water collection from fog. The multi-electrode structure consists of three parts: a charging electrode, an intercepting electrode and a ground electrode. Four types of water-collecting structures are compared experimentally, and the collection rates from a traditional fog mesh, a wire-mesh electrode with fog coming from a high-voltage electrode, a wire-mesh electrode with fog coming from a ground electrode and a multi-electrode structure are 2–3 g h-1, 100–120 g h-1, 60–80 g h-1 and 200–220 g h-1, respectively. The collection rate of the multi-electrode structure is 100‒150 times that of a traditional fog mesh and 2–4 times that of a wire-mesh electrode. These results demonstrate the superiority of the multi-electrode structure in fog collection. In addition, the motion equation of charged droplets in an electric field is also derived, and the optimization strategy of electrode spacing is also discussed. This structure can be applied not only to fog collection, but also to air purification, factory waste gas treatment and other fields.
Injection of solid hydrogen as well as its isotopes is a mature technology that has been applied in magnetic-confinement fusion devices for physical studies like plasma fueling, impurity transport, magnetohydrodynamic (MHD) instabilities and L-H mode transition since the 1980s [1, 2]. The technology has been widely applied in many fusion devices like EAST [3], DIII-D [4], KSTAR [5] and Tore Supra [6], and has been selected as a conventional fueling method for the International Thermonuclear Experimental Reactor (ITER) [7]. Compared with gas puffing, pellet injection has many advantages. For plasma fueling, pellet injection has higher fueling efficiency with deep particle deposition [8] and can improve plasma confinement [9]. Deep pellet injection can form a peaking density profile and improve the density limit [10, 11]. Moreover, the cryogenic pellet is a source of perturbation because it will cool the plasma abruptly and form localized high pressure around the plasmoid (a high-density low-temperature area of ablating pellet particles) during the pellet ablation phase. MHD activities have been seen during injection of pellets. Transient magnetic surface turbulence deduced by plasmoids has been detected and analyzed on TJ-II [12]. Continuous shallow pellet injection to the plasma edge has been applied to edge localized mode (ELM) control as the pellet can modulate the ELM's frequency and reduce the global heat load released by a burst of ELMs on the ASDEX Upgrade [13] and DIII-D [14]. Moreover, the study of pellet ablation and the density deposition process has also been carried out on other devices. The plasmoid will spread along the magnetic field line and meanwhile drift toward the low-field side (LFS) due to the ∇B effect [10, 15], and high-field side injection has been found to have higher fueling efficiency and deeper density deposition [10].
As presented, pellet injection is a widely used fueling and research method. The fueling methods on the Joint Texas Experimental tokamak (J-TEXT) are supersonic molecular beam injection [16] and gas puffing. To obtain a larger operation regime and satisfy more experiment requests, we have developed a pellet injection system on J-TEXT as a new fueling method. We describe the pellet injection system on J-TEXT in section 2. Then, the results of the experiments are presented in section 3. Finally, a summary is given in section 4.
A schematic of the pellet injection system on J-TEXT is shown in figure 1. The pellet injection system is composed of a pellet injector, a cryocooler/compressor system, gas circuits, a speed measurement chamber, a buffer chamber and electronic control systems. The working process is as follows. Pellets are formed in the pellet injector and launched by high-pressure He gas; the maximum pellet speed is about 310 m s-1. Then, the pellet flies through the diagnostic chamber and the pellet speed is measured by light gates using the time–distance method; a photograph of the pellet is taken by a video camera. Next, the pellets enter the buffer chamber; most of the H2, He gas that flows out with the pellets will stay in the buffer chamber and be pumped out. Finally, the lonely pellets will fly through the gate valve and enter the tokamak vacuum chamber.
The whole system is connected to the tokamak vacuum chamber from the LFS and perpendicularly to the plasma center, and the flight distance of the pellets from the injector to the plasma edge is about 4.5 m. This leads to a time delay of about 15‒21 ms for a normal pellet speed of 210‒310 m s-1. The pellet injector, buffer chamber and tokamak vacuum chamber are separated by a pneumatic valve and gate valve to facilitate independent tests.
A schematic diagram of the three-barrel pellet injector is shown in figure 2. It is provided by PELIN, LLC. To cool the injector and form pellets, a cryocooler is installed on the cylinder vacuum chamber of the injector and supplied by He circulation from the compressor. The cryocooler is a two-stage cryocooler: the first stage cooling power is 35 W, and the second stage cooling power is 5 W. The heat is transferred from the cold head, and it can decrease the cold-head temperature from room temperature to about ~7 K within 50 min. The whole cooling circulation is driven by the compressor that needs to work under a water-cooling circulation system. The bottom of the cryocooler, cold head and pellet barrels are all in the vacuum chamber that can maintain a vacuum environment of ~10-5 Pa via a molecular pump set to 110 l s-1 pump speed at high vacuum to prevent conduction heat loss. While in operation, aluminum foil wrapping around the cold head and cryocooler in the vacuum chamber is also used to reduce radiation heat dissipation. To converge the pellet barrels to the injection line, they are slightly bent after the cold head, as shown in figure 2, the bent angle is about 5° for the upper two barrels and about 12° for the lower barrel.
H2 is used to form the pellets because the fueling gas of the J-TEXT plasma is hydrogen and He is used as propellant gas because of its high sound velocity (~1000 m s-1). To form and launch pellets, H2 is supplied to three pellet barrels through a pipe and He is supplied through three independent solenoid fast valves to each barrel. When pellets are forming, fast valves are closed and H2 is supplied at a certain pressure. After the formation, the fast valve is triggered and high-pressure He will separate the pellet from the barrel wall and accelerate it.
In the J-TEXT SPI (shattered pellet injection) system [17], a mechanical punch is needed to expel the Ar and Ne pellet from the barrel wall because the shear strength of Ar and Ne is rather large at low temperature. But for the H2 pellet, the shear strength is lower; therefore, it can be broken away by the pressure of He. The pressure needed to expel the pellet from the barrel can be estimated as P=4σL/D, where σ is the shear strength, D is the pellet diameter and L is the pellet length [2]. P is about 0.32 MPa for a J-TEXT pellet of 1 mm length and 0.8 mm diameter, and the fast valve allows a highest output gas pressure of 0.8 MPa. The propellant gas pressure range is 0.5‒1.5 MPa in the inlet pipe of the fast valve. Therefore, the actual output pressure range is about 0.5‒0.8 MPa to launch the pellet. Each shot of pellet will consume about 0.15‒0.2 MPa of He gas in the 40 cm3 pipes when the pressure is above 1 MPa; therefore, the He gas release is about 6‒8 MPa·cm3 for launching each pellet. Therefore, it can perform continuous shots of three pellets, all with relatively stable maximum speeds. The dependence between pellet speed and propellant gas pressure in the experiments is shown in figure 3. The pellet speed can be adjusted by the propellant gas pressure, and the maximum speed is about 210‒310 m s-1 for He pressure above 0.8 MPa.
The cold head is at the end of the cryocooler that is directly connected to the pellet formation cells in the vacuum chamber of the injector. Figure 4 shows the structure of the cold head and barrels with pellet formation cells. Copper squares mechanically connect the cold head for thermal conduct and the barrels are welded in the coppers. Pellets are formed simultaneously by feeding hydrogen into stainless steel barrels, short sections of which are cooled by copper formation cells attached to the cooler cold head. The three barrels are connected with the three fast valves, respectively, and the H2 pipe together. Each inner diameter of the barrels is 1 mm, 0.8 mm and 0.8 mm. The distance between each barrel is about 25 mm. Thus, the diameter of a pellet is the same as the diameter of the barrel. Each pellet contains 2.1 (3.2) × 1019 atoms for 0.8 mm (1 mm) diameter with 0.8 mm length. A low-temperature sensor is attached to the cold head to measure the temperature. There are four heaters: one is inside the cold head to adjust the cold-head temperature, the other three are winded around the pellet barrels to produce barrel heating. When forming pellets, the cold-head temperature will be adjusted to about 9‒11 K by heaters inside the cold head, and the heaters on the barrels can control the length of pellets to about 0.8 mm fixed. H2 gas pressure is controlled and supervised at 0.05‒0.06 MPa to form pellets via gauges and a pressure valve in the gas circuits near the barrels. The pellets are formed in 3‒4 min, which is the same as the discharge time interval on J-TEXT.
A gas supply system including H2 and He gas cylinders and gas circuits can provide fueling and propellant gas to the injector, as shown in the dashed box of figure 1. The gas circuit is connected to the injector by three fast valves to transmit He and a single pipe to transmit H2. The gas circuits have pipes/valves and gauges that can control the transmission of H2 and He to the pellet injector, including gas filling and pumping, and are controlled by the electrical control system. For the H2 supply, a receiver is used to stabilize the H2 pressure when forming pellets. The mechanical pump with 4 l s-1 pump speed connected at the bottom of the gas circuits and the outlet of the pellet injector can pump out the gas from the back and front of the pellet barrels in the pellet injector for pellet formation and the launch process.
The diagnostic chamber after the pellet flies out of the injector can measure the pellet speed and take photographs of the pellet, as shown in figure 5. It is composed of a tunnel, which the pellet flies through, two pairs of light gates and a video camera. The light gate has a photo detector and a light emission diode. When a pellet flies pass the photo detector, the LED light will be partly shadowed and the output signal of the detector will change and generate a pulse. The pellet speed is measured using a time–distance method, which depends on the distance and pulse time interval of the two light gates. Meanwhile, the video camera lit by the flash lamp will take continuous photographs and capture a photograph of the flying pellet.
The injector operates under the control of a PC/PLC (Programmable Logic Controller) system (figure 6). The PLC provides low-level control—acceleration timing, temperature stabilization and pressure setting. The PC provides the operator interface and high-level control—formation sequence. All the information about the operation of the injector, such as temperature and heater power, H2 and He pressure and the state of the valves, as well as the settings, is transmitted via a USB port. The cold-head temperature control uses the proportion integral derivative (PID) regulator on the PLC level. The measured temperature signal is fed to the PID regulator, and pulse modulation is used to control the heaters. Fuel and pressures are controlled by input and exhaust valves in the gas supply system with small hysteresis. If the pressure is lower than the set value, the input valve will open and, if the pressure is higher than the set value, the exhaust valve will open. The fast valves can be triggered by both the PLC and the trigger system: the former is for bench testing, and the latter is for experiments. The pulse length of the fast valves is also controlled by the PLC.
When the pellets are injected, the propellant gas He will also flow out of the pellet barrels into the guide tube. Therefore, to prevent gas from entering the plasma, pellets will fly through a big buffer chamber (shown in figure 7), and most of the gas will stay in the buffer chamber and be pumped out. The buffer chamber has two stages. Each buffer stage has 40 l volume and is connected by the guiding tube, and both are connected to the same molecular pump set at a pumping speed of 600 l s-1 at high vacuum and the lowest pressure of ~4×10-6 Pa. Instead of using one large pump set, the two-stage buffer chamber can also be pumped by differential pumping of two pump sets to get better gas prevention. The volume of He pipes is about 40 cm3 with 0.5‒1.5 MPa pressure, and each launch of three pellets will inject ~18‒24 MPa·cm3 He. The pressure in the first buffer chamber will increase to no more than 400 Pa with continuous injection of three pellets at maximum initial pressure near 1.5 MPa and, at the same time in the second buffer chamber, the pressure is less than ~10-2 Pa. Experiment shots were also carried out by launching without forming a pellet, and almost no gas was found to enter the tokamak vacuum chamber.
The guide tubes in the buffer chamber are transited with gaps. When a pellet flies through the gaps, there will be deviation due to gravity and mechanical error. Therefore, the tubes' inner diameters are designed to be 8 mm, 10 mm, 12 mm and 14 mm, which are much larger than the pellet diameter, and are increased in stages to ensure that the pellets can enter the next stage. Between the guide tubes, the gap lengths of gap1 and gap2 are 20 mm, while gap3 is 40 mm because of the gate valve. The buffer chamber is connected between the speed measure module and the tokamak vacuum chamber, and the gate valve is separating them if a system test is needed.
A bench test was carried out after the installation of the system. Figure 8 shows a photograph of the pellet passing through the diagnostic chamber taken by the video camera, which checks the intactness of the pellet. Also, to test if the pellets can get out of the tubes in the buffer chamber, tests were carried out by hitting the aluminum foil of 0.1 mm thickness placed at the outlet of the tube (shown in figure 9). The impact force of the pellet is weak, and a single pellet cannot penetrate the foil but leaves a pit. The tests ensure the entrance of pellets into the tokamak vacuum chamber and, from the bench tests, the generation and launch success rate of the pellets is > 90%.
After design and installation of the system, the first pellet injection experiments were carried out in ohmic heating and limiter configuration discharges on J-TEXT. J-TEXT is a conventional tokamak with major radius R=1.05 m and minor radius a=25‒29 cm, changeable by position of the limiter [18, 19]. The plasma volume is about ~1.3 m3 and the line-averaged plasma density is in the range of ne=(1‒6) × 1019 m-3. The maximum plasma current and toroidal magnetic field are Ip=230 kA and Bt=2.3 T. The pulse length is about 600 ms.
As figure 10 shows, the pellet injection system is at an equatorial port of the J-TEXT tokamak on the LFS. Relevant diagnostics are also shown in figure 10. The fast camera can capture plasma visible radiation during the pellet injection at 20000 fps. The three-wave far infrared (FIR) laser polarization interferometer system (POLARIS) arranged with 17 vertical chords is used to measure line-integrated plasma density in the range r = -24 cm to r=24 cm with 1.5 cm chord interval and < 1 μs time resolution [20]. The 24-channel heterodyne electron cyclotron emission (ECE) radiometer, covering most of the plasma within the frequency range of 80–125 GHz, is used for relative electron temperature measurement [21]. Hα (656 nm) [22], soft X-ray (SXR) [23] and absolute extreme ultraviolet (AXUV) [24] arrays on ports 3 and 4 can measure corresponding radiation. In addition, a diamagnetic loop system is used to measure the plasma energy [25].
Figure 11 shows a photograph of an intact pellet captured by CCD camera with a CIII optical filter. It is shown that the pellet flies through the mid-plane from port 10. A typical shot of pellet fueling is shown in figure 12. The plasma current Ip=180 kA and the toroidal magnetic field Bt=1.8 T. In this shot, two pellets of 0.8 mm diameter are triggered with a 50 ms time interval. After pellet injection, the density steeply increases first and then gradually increases. The plasma energy gradually improves with density. The energy is calculated from the diamagnetic loop. Its value and unit is (c+b) kJ, where c is the absolute value. But, because it is difficult to calibrate precisely, the figure shows an uncalibrated value with a deviation b. During pellet injection, the loop voltage and plasma current do not change apparently. Therefore, the ohmic heating power is steady at about 200 kW, and the plasma energy improves about 1.5‒2 kJ in total. The energy confinement time τE=W/P improves by about 7.5‒10 ms. The plasma temperature drops immediately when the pellet enters the plasma, which shows fast cooling of the plasma by the pellet; then, the temperature recovers to pre-pellet level instead of remaining at a low level. The SXR and AXUV emission drops first but then rises, and exceeds the value in the pre-pellet phase. The plasma displacement dx always increases for a moment, which means the plasma moves toward the LFS horizontally.
The pellet fueling efficiency was estimated. The efficiency is defined by (n2 - n1)/np, where n2 is the plasma particle containment at the time when the core line-averaged density reaches the maximum value, n1 is the plasma particle containment several milliseconds before pellet injection, and np is the pellet H atom containment. The plasma particle containment is calculated by
A H2 pellet injection system for LFS pellet injection was developed and tested on the J-TEXT tokamak. The system includes a pellet injector, a gas supply system, a control system, a cryocooler as a cooling system and a buffer chamber. A three-barrel pellet injector is used to generate solid H2 pellets with diameters of 0.8 mm/0.8 mm/1 mm and length of 0.8 mm. The number of atoms in the pellet is 2.1×1019 for 0.8 mm diameter and 3.2×1019 for 1 mm diameter. The pellet is accelerated by high He at 0.5‒1.5 MPa gas pressure, and the pellet speed can reach 310 m s-1. Via injection of pellets, improved plasma confinement and increased plasma energy is found. The fueling efficiency is about 20%‒50% in ohmic heating plasma. In the future, more experiments and further detailed data analysis will be carried out.
This work is supported by the National Key Research and Development Program of China (Nos. 2016YFC0401002 and 2016YFC0401006), and National Natural Science Foundation of China (Nos. 51577080 and 51821005).
[1] |
Domen J K et al 2014 Clean Technol. Environ. Policy 16 235 doi: 10.1007/s10098-013-0645-z
|
[2] |
Huang G et al 2021 Mater. Lett. 289 129424 doi: 10.1016/j.matlet.2021.129424
|
[3] |
Gao L et al 2021 Water Res. 195 116957 doi: 10.1016/j.watres.2021.116957
|
[4] |
Xu L Q et al 2021 Water Res. 190 116782 doi: 10.1016/j.watres.2020.116782
|
[5] |
Wahlgren R V 2001 Water Res. 35 1 doi: 10.1016/S0043-1354(00)00247-5
|
[6] |
Khalil B, Ouarda T B M J and St-Hilaire A 2015 Sust. Water Resour. Manage. 2 71 doi: 10.1007/s40899-015-0038-z
|
[7] |
Tu Y D et al 2018 Joule 2 1452 doi: 10.1016/j.joule.2018.07.015
|
[8] |
Cheng X D 2004 Studies on the character of corona discharge and the performance of fog-removal about wire-mesh electrode MSc Thesis Xi'an University of Architecture and Technology, Xi'an, China (in Chinese)
|
[9] |
Hering S V et al 1987 Environ. Sci. Technol. 21 654 doi: 10.1021/es00161a006
|
[10] |
Parker A R and Lawrence C R 2001 Nature 414 33 doi: 10.1038/35102108
|
[11] |
Zheng Y M et al 2010 Nature 463 640 doi: 10.1038/nature08729
|
[12] |
Ju J et al 2013 Adv. Mater. 25 5937 doi: 10.1002/adma.201301876
|
[13] |
Robert S et al 2001 10th IRCSA Conf. (Mannheim: Int. Rainwater Catchment System Association)
|
[14] |
de Dios Rivera J 2011 Atmos. Res. 102 335 doi: 10.1016/j.atmosres.2011.08.005
|
[15] |
Park K C et al 2013 Langmuir 29 13269 doi: 10.1021/la402409f
|
[16] |
Fessehaye M et al 2014 Renew. Sust. Energy Rev. 29 52 doi: 10.1016/j.rser.2013.08.063
|
[17] |
Carvajal D et al 2020 Atmos. Res. 245 105123 doi: 10.1016/j.atmosres.2020.105123
|
[18] |
Li J Q et al 2021 J. Colloid Interf. Sci. 581 545 doi: 10.1016/j.jcis.2020.07.153
|
[19] |
Fan H F and Guo Z G 2021 J. Colloid Interf. Sci. 591 418 doi: 10.1016/j.jcis.2021.01.076
|
[20] |
Ehrenmann J, Henninger S K and Janiak C 2011 Eur. J. Inorg. Chem. 2011 471 doi: 10.1002/ejic.201001156
|
[21] |
Kim H et al 2017 Science 356 430 doi: 10.1126/science.aam8743
|
[22] |
Wang J Y et al 2017 Energy 138 542 doi: 10.1016/j.energy.2017.07.106
|
[23] |
Luan P C and Jordan J B 1969 IEEE Trans. Geosci. Electron. 7 250 doi: 10.1109/TGE.1969.271359
|
[24] |
Cruzat D and Jerez-Hanckes C 2018 J. Electrost. 96 128 doi: 10.1016/j.elstat.2018.10.009
|
[25] |
Li J C et al 2021 Plasma Sci. Technol. 23 064010 doi: 10.1088/2058-6272/abf6ad
|
[26] |
Singh S et al 2018 IEEE Trans. Plasma Sci. 46 3031 doi: 10.1109/TPS.2018.2850803
|
[27] |
Dau V T et al 2018 J. Aerosol Sci. 124 83 doi: 10.1016/j.jaerosci.2018.07.007
|
[28] |
Choi H Y, Park Y G and Ha M Y 2020 J. Mech. Sci. Technol. 34 3303 doi: 10.1007/s12206-020-0722-2
|
[29] |
Gao W C et al 2020 Powder Technol. 361 238 doi: 10.1016/j.powtec.2019.08.046
|
[30] |
Damak M and Varanasi K K 2018 Sci. Adv. 4 eaao5323 doi: 10.1126/sciadv.aao5323
|
[31] |
Yan X D and Jiang Y 2021 Atmos. Res. 248 105251 doi: 10.1016/j.atmosres.2020.105251
|
[32] |
Johnson M J and Go D B 2017 Plasma Sources Sci. Technol. 26 103002 doi: 10.1088/1361-6595/aa88e7
|
[33] |
Yamamoto T and Velkoff H R 1981 J. Fluid Mech. 108 1 doi: 10.1017/S002211208100195X
|
[34] |
Yamamoto T et al 2008 IEEE Trans. Industry Appl. 45 2178 doi: 10.1109/TIA.2009.2031859
|
[35] |
Yang Y et al 2021 J. Phys. D: Appl. Phys. 54 175204 doi: 10.1088/1361-6463/abdefd
|
[36] |
Wang P Y et al 2020 Plasma Sources Sci. Technol. 29 045005 doi: 10.1088/1361-6595/ab7733
|
[37] |
Niu S J et al 2010 Adv. Atmos. Sci. 27 639 doi: 10.1007/s00376-009-8174-8
|
[38] |
Lu C S et al 2013 Acta Meteor. Sin. 27 832 doi: 10.1007/s13351-013-0610-0
|
[39] |
Li Q et al 2011 High Voltage Eng. 37 2506 (in Chinese) doi: 10.13336/j.1003-6520.hve.2011.10.004
|
[40] |
Zhao Y S and Zhang W L 2013 Proc. CSEE 33 194 (in Chinese) doi: 10.13334/j.0258-8013.pcsee.2013.13.019
|
[41] |
Chen L W et al 2020 Sep. Purif. Technol. 247 116964 doi: 10.1016/j.seppur.2020.116964
|
[42] |
Zhang M et al 2021 J. Phys. D: Appl. Phys. 54 255201 doi: 10.1088/1361-6463/abf0ef
|
[43] |
Chen S et al 2019 IET Sci. Meas. Technol. 13 1212 doi: 10.1049/iet-smt.2019.0032
|
[44] |
Bian X M et al 2010 Proc. CSEE 30 118 (in Chinese) doi: 10.13334/j.0258-8013.pcsee.2010.04.015
|
[1] | L MIAO, Yu M GRISHIN. Dynamic heating and thermal destruction conditions of quartz particles in polydisperse plasma flow of RF-ICP torch system[J]. Plasma Science and Technology, 2020, 22(11): 115505. DOI: 10.1088/2058-6272/abad1a |
[2] | Zeyu HAO (郝泽宇), JianSONG(宋健), YueHUA(滑跃), Gailing ZHANG (张改玲), Xiaodong BAI (白晓东), Chunsheng REN (任春生). Frequency dependence of plasma characteristics at different pressures in cylindrical inductively coupled plasma source[J]. Plasma Science and Technology, 2019, 21(7): 75401-075401. DOI: 10.1088/2058-6272/ab1035 |
[3] | Jianwu HE (贺建武), Longfei MA (马隆飞), Senwen XUE (薛森文), Chu ZHANG (章楚), Li DUAN (段俐), Qi KANG (康琦). Study of electron-extraction characteristics of an inductively coupled radio-frequency plasma neutralizer[J]. Plasma Science and Technology, 2018, 20(2): 25403-025403. DOI: 10.1088/2058-6272/aa89e1 |
[4] | Xiuquan CAO (曹修全), Deping YU (余德平), Yong XIANG (向勇), Chao LI (李超), Hui JIANG (江汇), Jin YAO (姚进). Study on the ignition process of a segmented plasma torch[J]. Plasma Science and Technology, 2017, 19(7): 75404-075404. DOI: 10.1088/2058-6272/aa62f9 |
[5] | Hiromichi TOYOTA, Otsuka KAZUHIKO, Hidekazu GOTO. One-step phenol production from a water– toluene mixture using radio frequency in-liquid plasma[J]. Plasma Science and Technology, 2017, 19(5): 55503-055503. DOI: 10.1088/2058-6272/aa5fc6 |
[6] | WANG Songbai (王松柏), LEI Guangjiu (雷光玖), BI Zhenhua (毕振华), H. GHOMI, YANG Size (杨思泽), LIU Dongping (刘东平). Saturation Ion Current Densities in Inductively Coupled Hydrogen Plasma Produced by Large-Power Radio Frequency Generator[J]. Plasma Science and Technology, 2016, 18(9): 907-911. DOI: 10.1088/1009-0630/18/9/06 |
[7] | YU Minghao(喻明浩), Yusuke TAKAHASHI, Hisashi KIHARA, Ken-ichi ABE, Kazuhiko YAMADA, Takashi ABE. Numerical Investigation of Flow Fields in Inductively Coupled Plasma Wind Tunnel[J]. Plasma Science and Technology, 2014, 16(10): 930-940. DOI: 10.1088/1009-0630/16/10/06 |
[8] | LIU Wenyao (刘文耀), ZHU Aimin (朱爱民), Li Xiaosong (李小松), ZHAO Guoli (赵国利), et al.. Determination of Plasma Parameters in a Dual-Frequency Capacitively Coupled CF 4 Plasma Using Optical Emission Spectroscopy[J]. Plasma Science and Technology, 2013, 15(9): 885-890. DOI: 10.1088/1009-0630/15/9/10 |
[9] | DAI Zhongling(戴忠玲), YUE Guang(岳光), WANG Younian(王友年). Simulations of Ion Behaviors in a Photoresist Trench During Plasma Etching Driven by a Radio-Frequency Source[J]. Plasma Science and Technology, 2012, 14(3): 240-244. DOI: 10.1088/1009-0630/14/3/10 |
[10] | D. S. RAWAL, B. K. SEHGAL, R. MURALIDHARAN, H. K. MALIK. Experimental Study of the Influence of Process Pressure and Gas Composition on GaAs Etching Characteristics in Cl2/BCl3-Based Inductively Coupled Plasma[J]. Plasma Science and Technology, 2011, 13(2): 223-229. |
1. | Wang, Z., Mao, X., Lou, Y. et al. Protective film on cerium metal through in-situ formation of a dense CeO2 oxide layer using air plasma. Chemical Engineering Journal, 2025. DOI:10.1016/j.cej.2024.158285 |
2. | Zhao, Y., Yue, X., Zhang, P. et al. Removal mechanism and cleaning method of electroplating masking paint with a microwave plasma jet. Journal of Manufacturing Processes, 2024. DOI:10.1016/j.jmapro.2024.07.020 |
1. | Wang, Z., Mao, X., Lou, Y. et al. Protective film on cerium metal through in-situ formation of a dense CeO2 oxide layer using air plasma. Chemical Engineering Journal, 2025. DOI:10.1016/j.cej.2024.158285 |
2. | Zhao, Y., Yue, X., Zhang, P. et al. Removal mechanism and cleaning method of electroplating masking paint with a microwave plasma jet. Journal of Manufacturing Processes, 2024. DOI:10.1016/j.jmapro.2024.07.020 |