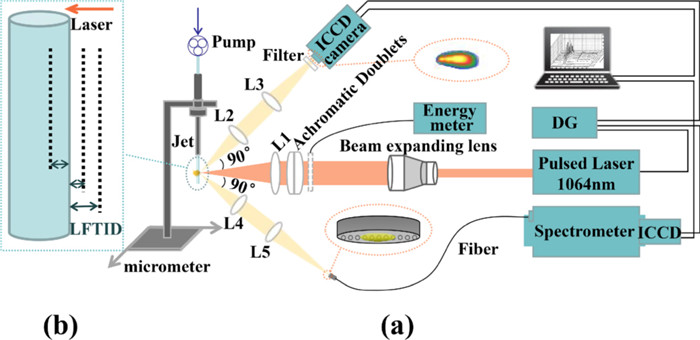
Citation: | Yuanyuan XUE, Mingda SUI, Runze LIU, Yiping WANG, Jinjia GUO, Ye TIAN, Jiamin LI, Meijie LIU, Shilei ZHONG, Gongyi XUE. Influence of the position relationship between gas–liquid interface and laser focus on plasma evolution characteristics in jet LIBS technology[J]. Plasma Science and Technology, 2023, 25(8): 085503. DOI: 10.1088/2058-6272/acc054 |
In order to understand the characteristics of breakdown process, plasma evolution and spectral emission in liquid jets laser-induced breakdown spectroscopy methods under the influence of the position variation between laser focus and gas–liquid interface, this work takes the plasma generated by laser-induced liquid jets as the object of study and discusses the changes in the spatial and temporal evolution characteristics and spectral radiation of the plasma when the position parameters between the laser focal point and the gas–liquid interface are different. The initial breakdown position is always between the front interface and the focus when the laser focus moves along the vertical direction of the interface, forming a phenomenon like 'interface effect'. The relationship between laser pulse energy and breakdown probability exhibits a law similar to a 'hysteresis curve' in the study of breakdown threshold. In this work, plasma with smaller size, rounder shape, stronger radiation, higher temperature, and higher density can be produced when the focus position is in the liquid column 0.2 mm away from the front interface. Simultaneously, the spectral signal intensity and signal-to-back ratio of the characteristic peaks of target elements in water reach maximum values, and the spectral signal becomes more stable (relative standard deviation value reaches 2%). The Ca element's ion radiation at 393.366 nm and atomic radiation at 422.673 nm are studied using narrow-band filtering imaging and time-space resolution spectroscopy. The findings demonstrate that the laws of ion and atomic radiation are not perfectly consistent in space and time.
Laser-induced breakdown spectroscopy (LIBS) is a technology for analyzing the elemental composition of samples that uses atomic emission spectroscopy. In this technology, focused short pulse width and high power density laser pulses are used to generate plasma by breaking through the sample, and the qualitative and quantitative detection of the element components in the sample is completed by analyzing the spectrum radiated by the element components in the sample when the plasma is cooled. LIBS technology has sparked widespread interest since its introduction in the 1960s [1] due to its fast, high sensitivity, simultaneous detection of multiple elements, and no sample pretreatment. The detection technology based on LIBS has advanced rapidly in metallurgy [2], minerals [3], atmosphere [4], soil [5, 6], medicine [7, 8], and other fields. LIBS has a promising future in the field of liquid sample detection. Its use in deep sea [9], environmental detection [4], industry [10], and other fields demonstrates LIBS technology's enormous potential.
However, the quality of the LIBS signal is affected by the matrix effect, energy absorption, high density, and other characteristics [11, 12], as well as the more complex plasma and shock wave generated by laser-induced liquid samples, resulting in poor sensitivity and stability in LIBS detection of liquid samples. Many new methods to improve LIBS signal of liquid samples have been proposed by domestic and foreign scholars. After the samples are converted into liquid columns or droplets by jet flow [13–15], liquid surface [16], liquid droplets [17], aerosol [18], micro gas column [19], and other methods, the method of LIBS detection can not only effectively improve the signal, but also maintain the inherent advantages of LIBS such as no sample processing, simultaneous multi-element detection, and fast real-time. As a result, it can be applied on-site, on-line, continuously, or even in situ.
The introduction of various forms of gas–liquid interface, which changes the interaction effect between laser and sample and thus improves their final detection ability, is a feature shared by these methods. Yaroshchyk et al [15] proposed a 1 mm diameter gas–liquid interface form of a vertical liquid jet. The atomic emission intensity was found to be strongly correlated with the laser pulse energy, focus position, and luminous volume of the liquid sample. The maximum emission signal position would shift along the beam propagation axis, and the amount of displacement would increase as laser energy increased. By converting liquid into droplet aerosol, Zhong et al [18] proposed that the ultrasonic nebulizer assisted LIBS (UN-LIBS) can maximize the gas–liquid interface. When the inside of the aerosol is chosen as the breakdown point, the detection limit of most elements of this method is one to three orders of magnitude lower than that of other LIBS signal enhancement methods, and it has good stability, with relative standard deviation (RSD) reaching 0.0076. Jiang et al [19] proposed a micro- gas column in liquid with a gas–liquid interface. The peak intensities of Mg, H, and Na elements were observed to be at a high level when the breakdown point was about 0.2 mm behind the gas–liquid interface, increasing the detection limit by an order of magnitude. Xue et al [20] investigated the secondary breakdown characteristics of double-pulse laser-induced breakdown spectroscopy (DP-LIBS) in water and discovered that by optimizing the axial focusing position and the laser energy of the second laser pulse, a stable plasma could be formed at the rear bubble-water interface and then expanded into the bubble to achieve strong spectral intensity and high repeatability, significantly enhancing the performance of DP-LIBS. It can be seen that when the liquid sample LIBS passes through the gas–liquid interface, it has an obvious enhancement effect, and the relationship between the focal point and the gas–liquid interface has an obvious influence on this signal enhancement effect.
According to physical mechanisms, the appearance of a gas–liquid interface causes various absorption and plasma evolution processes. The absorption of laser energy by the gas–liquid interface is obviously distinct from the breakdown in liquid, and it is also distinct from the breakdown in opaque solid. Surface transmission efficiency is low for solids, energy absorption and plasma generation occur primarily on the surface, and lens-to-sample distance (LTSD) has a significant influence on plasma generation, spectral radiation, and collected signal intensity [21]. The transmission and absorption of the LIBS detection system's focused light path near the gas–liquid interface is more complicated than the almost complete absorption at the gas–solid interface. Liquid transmittance is greater, and the absorption rate at the interface differs from that of liquid and air [22–24]. The determination of the focus position with the highest energy density will be more difficult. The distance between the laser focus and the gas–liquid interface of the liquid column sample on the side of the laser incident direction will be an important consideration at this point. In terms of breakdown threshold, there are different breakdown thresholds among liquid, interface and air [25–27]. Even if the absorbed energy is lower at the lower breakdown threshold, it is possible to breakdown and form plasma earlier. The initial generation position of plasma will have different characteristics due to the different characteristics of pressure [14], different space constraints of expansion, energy exchange [28] and thermal conductivity on both sides of gas and liquid, causing the evolution process of plasma to have different characteristics.
At the moment, the research mainly focused on the evaluation of detection ability, while the discussion of plasma physical properties mainly focused on the temperature and density of the whole plasma. However, there is still a lack of comprehensive understanding of research on the physical properties, spectral radiation properties, and theoretical basis of laser-induced gas–liquid interface breakdown and plasma evolution. The above studies will serve as the basis to explain the related physical mechanism and the enhancement mechanism of the related gas–liquid interface by LIBS method. In this work, taking liquid jets as samples, the influence of the relative position of laser focus on the gas–liquid interface on the physical and spectral radiation properties of plasma is studied in the LIBS experiment.
As shown in figure 1(b), a jet LIBS system with simultaneous acquisition of plasma images and spatially resolved spectra is set up in the laboratory. A Q-switched Nd: YAG laser (Beamtech, Dawa 200) at 1064 nm emits laser pulses with a pulse width of 6.46 ns and a spot diameter of 7.5 mm in this system. The laser's output pulse energy can be adjusted between 0 mJ and 207 mJ, with a stable value of less than 1%. A peristaltic pump at a speed of 120 ml min−1 steadily pushed liquid out of a metal needle with an inner diameter of 0.3 mm, forming a columnar jet liquid sample. LFTID (laser focus to the interface distance) is defined as the distance between the laser focus and the gas–liquid interface of the liquid column sample on the side of the laser incident direction, as shown in figure 1(a). A beam expander expands the laser beam before it is focused (3×). To achieve a better focus effect, the focus lens group consists of an achromatic doublet lens (f = 75 mm) and a flat-convex quartz lens (L1, f = 100 mm). The collection light path is divided into two paths: image collection and spectrum collection, with a 90° included angle between their optical axes and the optical axis of the focusing light path. The image light path through a lens group composed of two quartz flat-convex lens (L2, L3, f = 38.1 mm) images the plasma on an ICCD (Andor, i Star 334 T) photosensitive surface. To filter the laser, a 900 nm long-wave filter (FEL0900) is placed in front of the ICCD. All the plasma images in this paper are the average result of 10 single-shot plasma images taken by ICCD. The light path of spectrum collection consists of two quartz flat-convex lens (L4, L5, f = 38.1 mm), spatial resolution fiber (arranged in order by 19 200 μm quartz fiber cores), spectrometer (Andor, SR-500i; the number of grating line pairs include 300 lines mm−1 and 1200 lines mm−1) and ICCD detector (Andor, i star 334t; 1024 × 1024). The radiation of the plasma is imaged at the collecting end of the optical fiber by a lens group, and the radiation at different positions of the plasma is collected by different optical fiber cores on the collecting end, and is coupled into the spectrometer. The plasma spatial resolution spectrum signal recorded by the ICCD detector can be obtained after the spectrometer splits the signal of each fiber core. The laser trigger signal and the gate switch signal of detector are controlled by the pulse signal generator controls (Quantum Composers, 9520).
The sample used in this work is a standard seawater solution, which is prepared under laboratory conditions according to the contents of various elements in standard seawater [29] (1290 mg l−1 Mg, 412 mg l−1 Ca, 7.9 mg l−1 Sr, 10770 mg l−1 Na, and 399 mg l−1 K). The spectral data obtained primarily marked and processed the spectral signals of Ca, Mg, and H elements. Excel and Origin software are used to process the spectral data, and MATLAB software is used to draw the plasma cross-section view.
The images of the initial stage of plasma at different relative distances were taken in this paper to understand the influence of the relative position of the focal point and the gas–liquid interface on the position of plasma generation. During the shooting process, each plasma image is obtained with 5 ns delay, 5 ns gate width, and integration of 10 pulses after laser emission. Photographing an object of known size under the same light path arrangement determines the magnification of the imaging light path.
Using the gas–liquid interface of the liquid column sample as the initial position (LFTID = 0 mm) and the laser propagation direction as the positive direction, six different positions (denoted by ①–⑥) with LFTID values of 0.3 mm, 0.2 mm, 0.15 mm, 0.05 mm, −0.05 mm, and −0.3 mm were chosen for experimental research. Figure 2 depicts the original images obtained. Figure 2(b) depicts the relationship between the initial center position (dotted line) and six positions (dot chain line) of plasma generation in the plane determined by the optical axis of the laser and the central axis of the liquid column, using the plasma position corresponding to the point with the highest intensity in the image as the center.
Figure 2(a) shows that when the position of the liquid column remains constant, the plasma image generated changes with the change of position from ① to ⑥ (dot chain line), that is, with the gradual decrease of the LFTID. When the focal point, chain-dotted line, does not enter the liquid surface, the plasma image at position ⑤ moves more in the laser incidence direction than the plasma image at position ⑥, when the focal point, dot chain line enters the liquid column, the plasma image at position ④ moves to position ①, the centers of initially generated plasma gradually moves away from the direction of laser incidence, and the moving distance grows larger and larger. It is marked in the liquid column image taken with the ICCD lens in order to more intuitively observe the relative changes between the focus position and the plasma generation position. The plasma generation position, that is, the dotted line, is all concentrated on the side of the liquid column front interface, demonstrating a phenomenon like 'interface effect' as shown in figure 2(b).
The strongest point in the image of plasma is used to determine the plasma center position, and then compared to the LFTID value. Figure 2(c) depicts the end result. It is shown that the centers of the initially generated plasma at the breakdown point on the air or liquid sides both move towards the ai–liquid interface side, and the closer to the liquid the greater the moving distance. At the position ⑤, where the focal point is not in the liquid, the generation position of plasma enters the inside of the liquid column.
The breakdown probability at six different positions was measured to understand the influence of different LFTID values on the breakdown threshold. 300 pulses were measured at each position in the experiment, and the experimental results obtained by calculating the breakdown probability are shown in figure 3(a).
The breakdown threshold of each focus position is different, as shown in figure 3(a), and the image has a 'hysteresis curve' shape similar to that in a magnetic field. When the breakdown threshold is defined as the laser energy with a breakdown probability of 50%, the breakdown threshold of air is 24.5 mJ, the breakdown threshold at ④ and ⑤ ⑥ is approximately 25 mJ, similar with air, and the breakdown threshold at ③ ② ① is approximately 12 mJ less than air. The difference in energy between air and liquid is greater than 10 mJ, and it does not change gradually or continuously.
Light absorption and the breakdown threshold around the gas–liquid interface should be related to these phenomena. The absorption coefficient (the ratio of the original laser energy to the laser energy after passing through the object) of air is 10−5 m−1 [22], that of liquid is 101 m−1 [23], and that of the gas–liquid interface is much lower (10−4 m−1 [30]). As a result, even though the air absorption coefficient is small, the laser energy absorbed by the liquid side increases rapidly as the waist center moves from the air to the interface, and given the lower breakdown threshold of the liquid, we speculated that plasma is primarily generated at the interface at this time. As a result, when the focus is in the air, the position of plasma generation shifts to the interface side, as shown in figures 3(b) and (c). When the beam's waist completely enters the liquid, on one hand, due to the larger absorption coefficient of the liquid, the absorbed laser energy accumulates more on the side where the laser comes from; on the other hand, due to the refraction phenomenon at the interface during incident, the initial position of plasma formation will also move towards the interface, as shown in figure 3(d). This may explain the bilateral 'interface effect'. When the focusing system's center of the beam waist is far enough away from the interface, the air determines the breakdown threshold; as the beam waist approaches the interface, the higher absorption coefficient and smaller breakdown threshold cause the overall breakdown threshold to be determined by the liquid.
Images of the plasma evolution process were collected from a time delay of 5–1420 ns to observe the plasma evolution process with a gate width of 5 ns, as shown in figure 4.
As seen in figure 4(a), the scale and shape of the plasma produced at different locations are observed. The plasma produced in air is 'elongated' as shown in figure 4(b), with an aspect ratio close to 2. The transverse diameter of the plasma at locations ⑥ ⑤ gradually expands from 2 to 4 mm. What is noteworthy is that in the early stages of plasma formation, as shown in figure 4(c), the plasma divides into two smaller plasmas in the early stage of plasma formation, and then gradually combines into one plasma. When the focal point, however, is at the four positions ④ ③ ② ① inside the liquid, and the plasma aspect ratio is close to 1, which is approximately circular, as shown in figure 4(d). For all six data sets, the plasma gradually takes on a 'semicircle' shape in the later stages of time evolution, as shown in figure 4(e), i.e. the plasma tends to be flattened on the side close to the liquid.
The above phenomena have been theoretically studied and speculated. When the focus is biased to the air side, both sides of the optical axis with the laser beam waist as the center have high energy density distribution in a certain length, causing the induced plasma to be in an elongated shape. When the center of the beam waist is close to but not entering the gas–liquid interface, the laser energy density near the gas–liquid interface is smaller but the absorption coefficient is larger. The absorbed laser energy reaches the breakdown threshold of water and a plasma is induced. While on the air side, though the absorption coefficient is smaller in air, the laser energy density is larger. The absorbed energy can also reach the breakdown threshold of air that is needed to produce a plasma. That may be the reason why the plasma is appeared as two parts as observed in the first 70 ns. As the two parts of plasma expand in volume and interact with each other, they combine into an integrated one plasma gradually, as shown in figure 4(a) (⑤ ⑥). When the beam waist enters the liquid column, the stress is relatively uniform about 50 ns later. So the plasma expands uniformly in all directions, resulting in a smooth plasma, as shown in figure 4(a) (④ ③ ② ①). At a later stage of plasma formation, the resistance of plasma increases at each position due to the dense droplets on one side of the liquid column, while the force on the air side is lower, so the plasma gradually assumes a 'semi-circle' shape as time passes.
To determine the plasma image area and total radiation at various focal points, the plasma image area and total radiation are calculated and compared by selecting an image with a complete plasma image and a relatively stable time delay of 320 ns, as shown in figure 5. Among them, 10% of the strongest pixel signal intensity of the original plasma image is taken as the threshold (pixels higher than the threshold are regarded as effective pixels, and pixels lower than the threshold are regarded as the background without plasma image) [31], and the total number of pixels of the plasma image is counted to represent the size of plasma, which is referred as 'image area' for short.
It can be seen from figure 5 that with the change of focal positions ①–⑥, the total radiation intensity of plasma first increases and then decreases obviously, while the image area first decreases and then increases. At the focal point ②, the total radiation intensity is 4.0447 × 107, which is about twice the minimum value, while the image area is 730 858 pixels, which is the minimum value.
It demonstrates that when the focal point is at position ②, the plasma is generated near the center of the liquid column, and the force around it is relatively uniform, binds it to a smaller shape, so the plasma is the smallest, and the radiation is stronger, implying that the plasma distribution is more concentrated, which is more conducive to the collection of spectral signals in the system.
The spectra of four elements Ca, H, Mg, and Na were collected using FVB (Full Vertical Binning) and Multi-track spectrometers through spatially resolved optical fiber to study the spectral characteristics of plasma radiation. The spectra shown in figure 6 were obtained with a time delay of 300 ns and a gate width of 1 ms (300 lines mm−1 grating for Ca and H, and 1200 lines mm−1 grating for Mg and Na).
The temperature and density of different focal points were analyzed to better understand the influence of different focal points on the physical properties of plasma. Limited by the radiation properties of plasma induced by the liquid sample, only a few spectral lines can be used to calculate the plasma parameters, the three lines of Ca (two ion lines at 393.366 nm, 396.847 nm and one atomic line at 422.673 nm) were selected for the calculation of temperature in this work. The main purpose for calculating the temperatures of plasma generated at different focal points was to understand the changing trend of plasma with different LFTID. Therefore, rather than the absolute value of temperature, the authors prefer to show how the plasma temperature changes with the location of the focus. According to the [19, 30, 32] and the data of this paper, the selected spectral lines can show the trend cared about. The discussion of electron density follows the same principle.
As for atomic line, the electron temperature is calculated by the Boltzmann plot method as in equation (1), with the horizontal and vertical coordinates taken as E2, ln(I2λ2/A1g1) respectively
ln(Iij/giAij)=ln(ns/Us(T))−Ei/kT, | (1) |
where, I is the spectral line intensity; gi, the degeneracy of the energy level; Aij. the probability of transition from level i to j; ns, is the total density of particles in the s energy state in the plasma; Us (T), the distribution function of particles under the temperature T; Ei, the corresponding energy of the i energy level; k, the Boltzmann constant and T, the excitation temperature. While for ion spectral lines, the horizontal coordinate is equal to (2) and the vertical coordinate is equal to (3)
E+E1p−ΔE | (2) |
ln(I1λ1/A2g2)−ln((2(2πmek)/h3ne)T3/2), | (3) |
where, E1p is the ionization energy of low ionization order; ΔE, the correction of ionization energy in plasma due to the interaction of charged ions; λ, the wavelength of the transition; ne, the plasma electron density; me, the mass of the electron and h, the Planck constant. The corresponding points of the horizontal and vertical coordinates of the ion and atomic spectral lines are represented in the same coordinate system and the best-fit straight line is depicted with a slope of −1/kT, from which the plasma temperature T can be calculated [30].
The electron density was calculated using the Hα spectral line at 656.279 nm [33]. As in equation (4)
Ne=C(Ne,T)Δλ3/2s, | (4) |
where Δλs is the half-height full width of the spectral line and C (Ne, T) is a coefficient that is weakly correlated with Ne and T, specific values can be obtained by the [34, 35].
In figure 7, the calculated plasma temperature density at different focal points is shown.
As shown in figures 7(a) and (b), at the focal point ②, the temperature of plasma reaches 11 771 K, and the density reaches 5.810 23 × 1016 cm−3, which are all higher than those at other focal points.
It is possible that at position ②, the stress around the plasma is uniform, which improves laser energy absorption and thus ablation efficiency, raising the plasma's electron temperature. The density of particles per unit area inside the liquid is higher in this position than in others, so the density of plasma generated after laser breakdown is also higher. This is consistent with the plasma image phenomenon at position ②.
To observe the evolution of the physical properties of the plasma at different focal points over time, the spectrometer was set to a delay of 100 ns with a gate width of 200 ns.
Twenty sets of time-resolved spectral lines were acquired at 200 ns intervals and the plasma temperature was calculated as a function of time, as shown in figure 7(c).
As shown in figure 7(c), in the first 2200 ns, the temperature at position ② is higher than those at other focal positions, and it can keep a high temperature for a long time in a stable state, which is consistent with the fact that the plasma image at position ② is nearly circular, and it is also consistent with the minimum RSD at position ② calculated above.
Five focal points with strong spectral lines were chosen for discussion in order to study the radiation characteristics of plasma at different focal points, and the spectral intensity, signal-to-back ratio (SBR), and RSD values of 20 groups of spectral data of Ca Ⅱ 393.366 nm and Mg Ⅱ 279.553 nm were calculated, as shown in figures 8(a)–(e). The spatially resolved spectral signals collected by dividing the plasma into fifteen regions using spatially resolved optical fibers in the multi-track mode of the spectrometer are shown in figures 8(c) and (f) to understand the influence of different spatial positions of the plasma on the spectral signals.
It can be seen from figure 8 that the absolute intensity and signal-to-back ratio of elements in position ② reach the maximum value, the signal intensity and signal-to-back ratio of Ca element in position ② are 1.02–1.83 times those of other positions, and the signal intensity and signal-to-back ratio of Mg element in position ② are 1.26–1.55 times those of other positions. It can be seen from figures 8(b) and (e) that the RSD values of Mg and Ca elements at the focal point ② both reach 2%, that is, the spectrum here is in the most stable state. Figures 8(c) and (f) show that the spectral intensity of plasma at different focal positions is symmetrically distributed in the direction of the laser optical axis, increasing first and then decreasing. At position ②, the spectral intensity of the plasma near the central region is higher than those at other positions, which is consistent with the above image results.
To observe the evolution of the plasma spectrum over time and its lifetime, a delay time of 100 ns was set with a gate width of 200 ns. The time-resolved spectral intensity pictures of the elements at different focal positions were obtained at 200 ns intervals. The intensities of Ca, Mg, and H are the highest when the time delay is 300 ns (hereafter, 279.553 nm of Mg Ⅱ, 393.366 nm of Ca Ⅱ, and 656.279 nm of Hα are used), and the lifetimes of elements in different focal positions differ. According to the calculation, the lifetimes of elements Mg and Ca in position ② are the shortest, with 1.5 μs of Mg and 1.7 μs of Ca. The spectral intensity changes most rapidly at element position ②, in which Mg decreases by 70 000 in 2 μs, Ca decreases by 25 000 in 3 μs, and H decreases by 280 000 in 4 μs. However, at the same time, the change of element intensity at other focal positions is less than that at position ②, which indicates that the plasma generation time at position ② is concentrated.
According to the above two groups of data, the spectral signal intensity, signal-to-back ratio, stability, lifetime and other aspects will be affected by the focus position, and the best spectral data can be obtained at the position ②.
The ionic line strength of Ca element (Ca Ⅱ 393.366 nm) and atomic line strength (Ca Ⅰ 422.673 nm) at point ② were compared, and the ratio between them was calculated as shown in figure 9, to investigate the problem that the enhancement multiple of ionic line strength at the gas–liquid interface is much higher than that of atomic line [19] proposed by our research group in previous experiments.
Figure 9 shows that under the time-resolved result of 4000 ns, the ion line intensity of Ca element first increases, reaches a maximum value at 300 ns, and then rapidly decreases, whereas the atomic line intensity gradually decreases. Within a delay time of 200–2000 ns, the intensity of the ion line is 1.07–1.42 times greater than that of the atomic line. After 2000 ns, the intensity difference between atomic and ion lines approaches zero.
The focal point ② was chosen for research to explain the different changes of spectral ion line atomic line signals at the gas–liquid interface from the perspective of spatial resolution in combination with plasma images, and narrow-band neutral density filters with central wavelengths of 390 nm, 405 nm, and 420 nm were placed before ICCD to collect the ion line atomic line images and radiation background images of Ca elements. Following that, the plasma cross-section diagram shown in figure 10(a) is obtained by processing the filter's transmittance and the corresponding background removal method [36] (in which the pixel intensities that are negative after background removal are all marked as 0). The upper part of each group of images is a Ca ion line (Ca Ⅱ 393.366 nm) diagram, and the lower part is a Ca atom line (Ca Ⅰ 422.673 nm) diagram, with the spatial resolution collected using the same parameters.
The image peaks of atomic line and ion line gradually separate with time, as shown in figure 10(a), and the spectral peaks of the atomic line and the ion line also gradually separate with time, as shown in figure 10(b), which is consistent with the image. They all show a trend of the ion line moving to the right and the atomic line moving to the left.
According to the above rules, it is speculated that the ion line moves closer to the gas–liquid interface during plasma evolution, while the atomic line moves closer to the inside of the liquid column. As a result, the spectral signal of an ion line collected in a gas–liquid interface system is stronger than that of an atomic line, and the intensity of an ion line collected in a gas–liquid interface system is greater than that collected in water.
Based on the results of imaging and spectral experiments, the plasma generation and evolution process under different laser focuses around the gas–liquid interface and the effect of focus position on spectral signals are described and explained in this paper.
The results show that the gas–liquid interface has a significant influence on the position of laser-induced plasma in the LIBS experiment of liquid jet. Whether the focus is on the liquid or gas side, plasma generation always occurs between the focus and the interface, i.e. it tends to the side where LFTID is 0 mm. This phenomenon is analogous to the electromagnetic 'interface effect'. This could be due to the absorption coefficient and breakdown threshold differences between liquid and gas. The results of the breakdown threshold experiment also show a 'hysteresis curve' relationship between threshold energy and focus position. The plasma produced by different focal positions has different shapes and sizes during the plasma evolution process, as does the radiation intensity. When the focal point is 0.2 mm away from the interface in the liquid column (LFTID is 0.2 mm), plasma with a smaller size, rounder shape, stronger radiation, higher temperature, and higher density can be produced. In terms of light path collection, plasma is more advantageous as a spectral emission source when the scale is smaller, the radiation is stronger, the temperature is higher, and the density is higher. All spectral lines collected at this focal point are more stable than those collected at other focal points. Using the Ca ion line at 393.366 nm as an example, the RSD value reaches a minimum of 2%, and the spectral signal intensity and signal-to-back ratio are also superior to those at other focal points, being 1.02–1.83 times greater. The distribution of ion and atomic radiation in different focal positions varies, demonstrating the additional influence of the interface on plasma generation and radiation process. The related research results in this paper are important in better understanding the interaction mechanism between laser and gas–liquid interface samples and the evolution law of induced plasma, as well as in explaining the enhancement mechanism and improving the enhancement effect of various gas–liquid interface enhancement LIBS methods.
This work supported by Natural Science Foundation of Shandong Province (Nos. ZR201910290171 and ZR2019MD016), National Key Research and Development Program of China (No. 2016YFC0302101) and National Natural Science Foundation of China (No. 41976173).
[1] |
Radziemski L J 2002 Spectrochim. Acta B 57 1109 doi: 10.1016/S0584-8547(02)00052-6
|
[2] |
Zeng Q et al 2018 Spectrochimica Acta Part B: Atomic Spectroscopy 142 68 doi: 10.1016/j.sab.2018.01.011
|
[3] |
Feng J et al 2011 Anal. Bioanal. Chem. 400 3261 doi: 10.1007/s00216-011-4865-y
|
[4] |
Gallou G et al 2011 Aerosol Sci. Technol. 45 918 doi: 10.1080/02786826.2011.566899
|
[5] |
Gaudiuso R et al 2010 Sensors 10 7434 doi: 10.3390/s100807434
|
[6] |
Kumar R et al 2019 J. Appl. Spectrosc. 86 942 doi: 10.1007/s10812-019-00919-w
|
[7] |
Jaswal B B S and Singh V K 2015 Appl. Spectrosc. Rev. 50 473 doi: 10.1080/05704928.2015.1010206
|
[8] |
Knight A K et al 2000 Appl. Spectrosc. 54 331 doi: 10.1366/0003702001949591
|
[9] |
Fortes F J et al 2015 J. Anal. At. Spectrom. 30 1050 doi: 10.1039/C4JA00489B
|
[10] |
Guy M et al 2012 Metall. Anal. 32 6 (in Chinese)
|
[11] |
Thornton B et al 2015 Deep Sea Res. Part Ⅰ: Oceanogr. Res. Pap. 95 20 doi: 10.1016/j.dsr.2014.10.006
|
[12] |
Lazic V and Jovićević S 2014 Spectrochim. Acta B 101 288 doi: 10.1016/j.sab.2014.09.006
|
[13] |
Kumar A, Yueh F Y and Singh J P 2003 Appl. Opt. 42 6047 doi: 10.1364/AO.42.006047
|
[14] |
Ohba H et al 2014 Opt. Express 22 24478 doi: 10.1364/OE.22.024478
|
[15] |
Yaroshchyk P et al 2004 Appl. Spectrosc. 58 1353 doi: 10.1366/0003702042475592
|
[16] |
Adamson M et al 2007 Spectrochimica Acta Part B: Atomic Spectroscopy 62 1348 doi: 10.1016/j.sab.2007.10.022
|
[17] |
Archontaki H A and Crouch S R 1988 Appl. Spectrosc. 42 741 doi: 10.1366/0003702884429049
|
[18] |
Zhong S L 2012 Analysis of trace metal elements in aqueous solution samples using ultrasonic nebulizer assisted LIBS PhD Thesis Ocean University of China, Qingdao, China (in Chinese)
|
[19] |
Jiang L L et al 2021 Spectrochim. Acta B 177 106065 doi: 10.1016/j.sab.2021.106065
|
[20] |
Xue B et al 2019 Spectrochim. Acta B 151 20 doi: 10.1016/j.sab.2018.11.005
|
[21] |
Zhao S R and Yi X Y 2004 J. Baoding Teach. Coll. 17 20 (in Chinese)
|
[22] |
Yang X L, Li Y and Wang J B 2004 Laser J. 25 40 (in Chinese) doi: 10.1049/el:20046892
|
[23] |
Deng R R et al 2012 J. Remote Sens. 16 192 (in Chinese)
|
[24] |
Hansen A D A, Rosen H and Novakov T 1982 Appl. Opt. 21 3060 doi: 10.1364/AO.21.003060
|
[25] |
Yang X Y et al 2016 Opt. Express 24 13410 doi: 10.1364/OE.24.013410
|
[26] |
Tambay R and Thareja R K 1991 J. Appl. Phys. 70 2890 doi: 10.1063/1.349359
|
[27] |
Kennedy P K, Hammer D X and Rockwell B A 1997 Prog. Quantum Electron. 21 155 doi: 10.1016/S0079-6727(97)00002-5
|
[28] |
Dell'Aglio M et al 2017 J. Phys. D: Appl. Phys. 50 185204 doi: 10.1088/1361-6463/aa652a
|
[29] |
Chen M 2009 Chemical Oceanography (Beijing: China Ocean Press) (in Chinese)
|
[30] |
Fan J J et al 2014 Spectrosc. Spect. Anal. 34 3183 (in Chinese)
|
[31] |
Negre E et al 2016 Spectrochim. Acta B 122 132 doi: 10.1016/j.sab.2016.06.009
|
[32] |
Tian Y et al 2019 Anal. Chem. 91 13970 doi: 10.1021/acs.analchem.9b03513
|
[33] |
Griem H R and Barr W L 1975 IEEE Trans. Plasma Sci. 3 227 doi: 10.1109/TPS.1975.4316912
|
[34] |
Parigger C G, Dackman M and Hornkohl J O 2008 Appl. Opt. 47 G1 doi: 10.1364/AO.47.0000G1
|
[35] |
Griem H R 1968 Phys. Rev. 165 258 doi: 10.1103/PhysRev.165.258
|
[36] |
Motto-Ros V et al 2012 Spectrochim. Acta B 74–75 11 doi: 10.1016/j.sab.2012.07.007
|
[1] | Hyun-Su JUN, Yat Fung TSANG, Jae Ok YOO, Navab SINGH. Theoretical study of particle and energy balance equations in locally bounded plasmas[J]. Plasma Science and Technology, 2024, 26(12): 125404. DOI: 10.1088/2058-6272/ad7df3 |
[2] | Liuxiu HE (何柳秀), Minghai LIU (刘明海), Shuangyun ZHAO (赵双云). Spontaneous magnetic field multipolar structure in toroidal plasmas based on 2D equilibrium[J]. Plasma Science and Technology, 2019, 21(4): 45101-045101. DOI: 10.1088/2058-6272/aaf78d |
[3] | Yang LIU (刘杨), Yue TONG (佟悦), Ying WANG (王莹), Dan ZHANG (张丹), Suyu LI (李苏宇), Yuanfei JIANG (姜远飞), Anmin CHEN (陈安民), Mingxing JIN (金明星). Influence of sample temperature on the expansion dynamics of laser-induced germanium plasma[J]. Plasma Science and Technology, 2017, 19(12): 125501. DOI: 10.1088/2058-6272/aa8acc |
[4] | Yong WANG (王勇), Cong LI (李聪), Jielin SHI (石劼霖), Xingwei WU (吴兴伟), Hongbin DING (丁洪斌). Measurement of electron density and electron temperature of a cascaded arc plasma using laser Thomson scattering compared to an optical emission spectroscopic approach[J]. Plasma Science and Technology, 2017, 19(11): 115403. DOI: 10.1088/2058-6272/aa861d |
[5] | Jianxun LIU (刘建勋), Yanyun MA (马燕云), Xiaohu YANG (杨晓虎), Jun ZHAO (赵军), Tongpu YU (余同普), Fuqiu SHAO (邵福球), Hongbin ZHUO (卓红斌), Longfei GAN (甘龙飞), Guobo ZHANG (张国博), Yuan ZHAO (赵媛), Jingkang YANG (杨靖康). High-energy-density electron beam generation in ultra intense laser-plasma interaction[J]. Plasma Science and Technology, 2017, 19(1): 15001-015001. DOI: 10.1088/1009-0630/19/1/015001 |
[6] | CHANG Lei (苌磊), LI Qingchong (李庆冲), ZHANG Huijie (张辉洁), LI Yinghong (李应红), WU Yun (吴云), ZHANG Bailing (张百灵), ZHUANG Zhong (庄重). Effect of Radial Density Configuration on Wave Field and Energy Flow in Axially Uniform Helicon Plasma[J]. Plasma Science and Technology, 2016, 18(8): 848-854. DOI: 10.1088/1009-0630/18/8/10 |
[7] | JIN Yizhou (金逸舟), YANG Juan (杨涓), TANG Mingjie (汤明杰), LUO Litao (罗立涛), FENG Bingbing (冯冰冰). Diagnosing the Fine Structure of Electron Energy Within the ECRIT Ion Source[J]. Plasma Science and Technology, 2016, 18(7): 744-750. DOI: 10.1088/1009-0630/18/7/08 |
[8] | BAI Yujing (白玉静), LI Jianquan (李建泉), XU Jun (徐军), LU Wenqi (陆文琪), WANG Younian (王友年), DING Wanyu (丁万昱 ). Improvement of the Harmonic Technique of Probe for Measurements of Electron Temperature and Ion Density[J]. Plasma Science and Technology, 2016, 18(1): 58-61. DOI: 10.1088/1009-0630/18/1/10 |
[9] | SUN Jiang (孙江), SUN Jianfeng (孙剑锋), YANG Hailiang (杨海亮), ZHANG Pengfei (张鹏飞), et al.. Plasma Density Influence on the Properties of a Plasma Filled Rod Pinch Diode[J]. Plasma Science and Technology, 2013, 15(9): 904-907. DOI: 10.1088/1009-0630/15/9/14 |
[10] | Y. YOSHIMURA, S. KUBO, T. SHIMOZUMA, H. IGAMI, H. TAKAHASHI, M. NISHIURA, S. OGASAWARA, R. MAKINO, T. MUTOH, H. YAMADA, A. KOMORI. High Density Plasma Heating by EC-Waves Injected from the High-Field Side for Mode Conversion to Electron Bernstein Waves in LHD[J]. Plasma Science and Technology, 2013, 15(2): 93-96. DOI: 10.1088/1009-0630/15/2/02 |