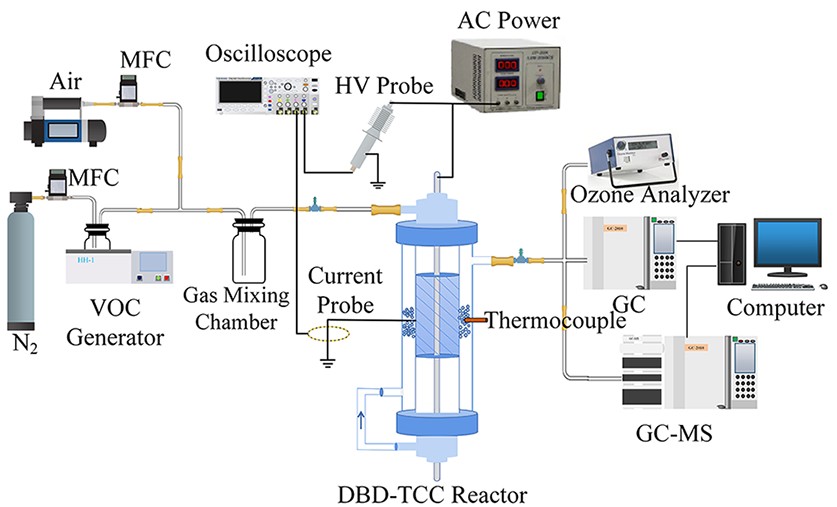
Citation: | Yue LI, Nan JIANG, Zhengyan LIU, Liang QIN, Bangfa PENG, Ronggang WANG, Yurong SUN, Jie LI. Efficient activation of the Co/SBA-15 catalyst by high-frequency AC-DBD plasma thermal effects for toluene removal[J]. Plasma Science and Technology, 2024, 26(8): 085502. DOI: 10.1088/2058-6272/ad47db |
Dielectric barrier discharge (DBD) plasma excited by a high-frequency alternating-current (AC) power supply is widely employed for the degradation of volatile organic compounds (VOCs). However, the thermal effect generated during the discharge process leads to energy waste and low energy utilization efficiency. In this work, an innovative DBD thermally-conducted catalysis (DBD-TCC) system, integrating high-frequency AC-DBD plasma and its generated thermal effects to activate the Co/SBA-15 catalyst, was employed for toluene removal. Specifically, Co/SBA-15 catalysts are closely positioned to the ground electrode of the plasma zone and can be heated and activated by the thermal effect when the voltage exceeds 10 kV. At 12.4 kV, the temperature in the catalyst zone reached 261 °C in the DBD-TCC system, resulting in an increase in toluene degradation efficiency of 17%, CO2 selectivity of 21.2%, and energy efficiency of 27%, respectively, compared to the DBD system alone. In contrast, the DBD thermally-unconducted catalysis (DBD-TUC) system fails to enhance toluene degradation due to insufficient heat absorption and catalytic activation, highlighting the crucial role of AC-DBD generated heat in the activation of the catalyst. Furthermore, the degradation pathway and mechanism of toluene in the DBD-TCC system were hypothesized. This work is expected to provide an energy-efficient approach for high-frequency AC-DBD plasma removal of VOCs.
The emission of volatile organic compounds (VOCs), primarily originating from fossil fuels and transportation activities, has led to environmental and human health issues, such as the greenhouse effect, photochemical smog, and respiratory ailments [1, 2]. To address the challenges posed by VOCs, various technologies have been established, including adsorption [3], condensation [4], membrane separation [5], catalytic oxidation [6], and non-thermal plasma (NTP) [7]. Among these, NTP is considered one of the most efficient strategies, as high-energy reactive species can rapidly decompose VOCs [8, 9]. When utilizing NTP technology for the treatment of VOCs, various discharge forms are available, such as dielectric barrier discharge (DBD) [10], gliding arc discharge [11], and corona discharge [12]. Specifically, DBD is the formation of low-temperature plasma between electrodes separated by a dielectric layer under an applied voltage. It exhibits superior degradation effects on VOCs due to its ability to generate high-energy electron density and rich content of active substances [13]. Furthermore, DBD plasma excited by a high-frequency alternating-current (AC) power supply has been extensively researched and applied in the process of VOC abatement as a mature and stable technology [14].
However, in high-frequency AC-DBD, a notable portion of the energy is dissipated as heat, resulting in low energy utilization efficiency [15]. Research on the thermal effects of AC-DBD reactors suggests that the heat released during the DBD process induces dielectric heating due to dielectric hysteresis, leading to significant heat energy consumption [16]. Nguyen and Lee [17] reported that the dielectric temperature in an AC-DBD reactor increased from room temperature to 460 °C after 15 min treatment at a frequency of 30 kHz and a voltage of 7 kV. In the work of Wang et al [18], approximately 72.5% of discharge energy was converted into heat when the DBD reactor was driven by a 9.5 kHz AC power supply at 11 kV. Li et al [19] employed high-frequency AC-DBD to remove toluene at an input energy of 10 kJ/L, achieving a toluene degradation efficiency of 73.6%. However, the energy efficiency was only 0.15 g/kWh due to light and thermal losses. Therefore, making effective use of the heat generated by high-frequency AC power is essential for minimizing energy waste and reducing the energy consumption of the plasma system. Moreover, after individual NTP treatment, the presence of untreated VOCs and intermediate byproducts remains, and low mineralization rates also pose a challenge [20].
The catalytic oxidation process is an effective method for treating VOCs with high mineralization rates. However, additional heating of the catalyst is helpful for achieving optimal efficiency [21]. Noble metal oxides serve as highly efficient catalysts for VOC degradation. Nevertheless, the drawbacks of these catalysts are evident, primarily in terms of their high cost and susceptibility to poisoning [22]. Consequently, many other non-precious metal oxides, such as Co3O4, MnO2, CeO2, and NiO [23, 24], have garnered attention and have been applied in the field of VOC removal. Among them, cobalt-based catalysts have been widely used in the catalytic oxidation of VOCs due to their superior low-temperature redox capacity, associated with the abundant mobile oxygen present in their structure [25, 26]. Ren et al [27] prepared a three-dimensional (3-D) Co3O4 catalyst that showed excellent low-temperature activity in the catalytic oxidation of toluene, with a T90% value of 238 °C. Bai et al [28] prepared porous Co3O4 nanorods and achieved a T90% value of 215 °C. Furthermore, by incorporating a molecular sieve carrier with a high specific surface area and uniform pore size, the limitation of low specific surface area in pure Co3O4 is addressed while simultaneously maintaining the low-temperature activity of the catalyst. Wen et al [29] investigated the catalytic oxidation of toluene using Co-based catalysts supported on different molecular sieves (KIT-6, SBA-15, SiO2). The results indicated that OM-Co3O4@SBA-15 exhibited the highest activity, with a T90% value of 260 °C. Nevertheless, it is crucial to note that the external heating required in the catalytic oxidation process represents a significant source of high energy consumption in this method. Therefore, if the heat generated during the high-frequency AC-DBD process can be utilized to activate the catalyst in the catalytic oxidation, it would greatly enhance plasma utilization efficiency, reduce energy waste, and improve the degradation effects of toluene. To the best of our knowledge, limited research has been conducted on the study of the catalytic activation of catalysts using the heat generated from AC-DBD to remove VOCs, and the degradation pathway of VOCs combining plasma with thermal catalysis is not clearly explored.
In this work, a novel DBD thermally-conducted catalysis (DBD-TCC) system has been proposed for toluene degradation. The DBD-TCC reactor adopts a dual-layer composite structure, with internal plasma discharge and external catalyst heat absorption. The objective is to utilize the thermal effects generated by the AC-DBD plasma to activate the catalyst and enhance energy efficiency. Firstly, the optimal cobalt-doping amount of the Co/SBA-15 catalyst in the DBD-TCC system was investigated and characterized, along with optimization of the space velocity. Moreover, the role of AC-DBD thermal effects in the activation of the Co/SBA-15 catalyst was examined by comparing the toluene degradation efficiency, CO2 selectivity, and energy efficiency among the DBD, DBD thermally-unconducted catalysis (DBD-TUC), and DBD-TCC systems. Furthermore, the stability of the Co/SBA-15 catalyst was verified by characterizing the catalyst before and after discharge in the DBD-TCC system. Additionally, the possible toluene degradation pathway and mechanism in the DBD-TCC system were analyzed by examining the gas-phase degradation products from both the DBD and DBD-TCC systems.
A schematic diagram of the DBD-TCC system experimental setup is shown in figure 1, which contains a DBD-TCC reactor driven by a high-frequency AC power supply (CTP-2000 K/P, 0–20 kV, 5–25 kHz), a continuous-flow toluene supply system, and electrical and gas analysis systems. The experiments were conducted under a fixed power supply frequency of 5 kHz. The gas flow rate was controlled using mass flow meters (MFC, D07-7, Beijing Xiaotao Technology, China). Here, 0.7 L/min N2 blew out the toluene placed in an ice bath, which was mixed with air generated by an air generator (W58D, Shanghai Fuhong, China) producing 2 L/min of air, finally producing toluene gas at a concentration of 200 ppm. An atmospheric pressure balancing pump was used at the reactor outlet after filling the catalyst to keep the inlet and outlet flow rates consistent. The gaseous products were detected at the inlet and outlet of the DBD-TCC reactor.
Voltage and current waveforms were measured using a voltage probe (P6015A, Tektronix, USA) and a current transformer (P6021A, Tektronix, USA), respectively. A digital phosphor oscilloscope (DS1104, RIGOL Technology, China) was used to record the waveforms of the discharge voltage and current. The Lissajous method was used to calculate the discharge power. The concentrations of toluene and CO2 were measured separately using gas chromatography (GC-2010, Shimadzu, Japan; GC7900, Techcomp, China), and O3 was monitored by an ozone analyzer (Model 106, 2B Technologies, USA). The gas was introduced into a CH2Cl2 solution, and the degradation products were detected using GC-MS (GC-7890A, MS-5975C, Agilent, USA). The toluene degradation efficiency (ηtoluene), CO2 selectivity (Sco2), and energy efficiency (ηeff) are defined as follows:
ηtoluene=(Cin−CoutCin)×100%, | (1) |
Sco2=Coutco2−Cinco27×(Cin−Cout)×100%, | (2) |
ηeff(g/kWh)=Cin×ηtoluene×M×Q×60P×22.4×100×10−3, | (3) |
where Q is the gas flow rate (L/min); Cin and Cout are the toluene concentrations at the inlet and outlet (ppm), respectively; Cinco2 and Coutco2 are the inlet and outlet concentrations of the CO2 (ppm), respectively; and M represents the relative molecular mass of toluene (g/mol).
In the thermal catalytic oxidation system, the reactor, toluene supply system, and gas analysis systems were configured identically to those in the DBD-TCC system. An additional tube furnace (SK2-2-12, XianKe Technology, China) was introduced to heat the catalyst region within the DBD-TCC reactor. The temperature selected in the thermal catalytic oxidation experiment is the same as the stable temperature that can be achieved in the catalyst region in the DBD-TCC system under different voltages. The specific thermal catalytic oxidation system experimental setup is shown in figure S1.
For comparison, in addition to the DBD-TCC system, the DBD system and DBD-TUC system were set up, as illustrated in figure 2. The experimental conditions in the three systems are the same, except for the reactor design. To ensure a consistent path for toluene gas within all three reactors, each reactor is constructed with inner and outer quartz tubes, both measuring 200 mm in length. The inner diameters of the inner and the outer tubes are 13 mm and 25 mm, respectively, with a thickness of 2 mm. The inner region of the inner tube serves as the plasma discharge area. A 5 mm diameter stainless-steel rod, functioning as the high-voltage electrode, is centrally positioned within the inner tube, while the ground electrode is an 80 mm stainless-steel mesh tightly attached to its outer layer. Two small branch tubes are placed on each side of the reactor outer tube, each 30 mm from the top and bottom tube openings. The mixed toluene initially passes through the inner tube plasma discharge area and then exits through the interlayer region between the inner and outer tubes.
In the DBD-TCC reactor (figure 2(b)), the interlayer area between the outer tube and the inner tube is filled with Co/SBA-15 catalyst. The catalysts are closely positioned adjacent to the dielectric tube and the ground electrode in the plasma discharge area, enabling them to absorb heat generated during the plasma discharge process. The mixed toluene undergoes initial passage through the plasma discharge zone before entering the Co/SBA-15 catalyst-filled zone. Temperature measurements are obtained using a thermocouple inserted into the catalyst zone. In the DBD-TUC reactor (figure 2(c)), the plasma discharge zone and the catalyst zone are separated, preventing the catalyst zone from conducting heat from the plasma discharge.
Here, 6.0 g of polypropylene-polyoxyethylene copolymer solution was dissolved in 90 mL of deionized water. Subsequently, 30.3 mL of 37% concentrated HCl was added, followed by slow dropwise addition of 13.6 mL of tetraethyl orthosilicate at 40 °C with continuous stirring. The resulting mixture was further stirred for 24 h and transferred to a reaction vessel for a 24 h hydrothermal crystallization reaction at 100 °C. After three cycles of filtration and centrifugation washing, the material was dried at 100 °C for 24 h. Finally, the white SBA-15 molecular sieve powder was calcined at 550 °C for 6 h to remove the template agent. Next, 5%, 10%, 15%, and 20% dosage ratios of Co (NO3)2·6H2O were weighed and thoroughly stirred with deionized water for 2 h to prepare a 15 mL mixture solution. Subsequently, the SBA-15 carrier was immersed in the solution for 24 h. The resulting mixture was then dried at 100 °C and finally calcined at 500 °C for 6 h to obtain the Co/SBA-15 catalyst.
The chemical composition and crystalline structure of the specimens were analyzed using X-ray diffraction (XRD, SmartLab 9 kW, Rigaku Corporation) utilizing Cu Kα radiation within the range of 20°–70° (2θ). Morphological examinations were conducted using scanning electron microscopy (SEM, JSM-7900F, JEOL). X-ray photoelectron spectroscopy (XPS) data were acquired using a Thermo ESCALAB 250X. Fourier transform infrared (FT-IR) spectroscopy was conducted using a Nicolet 6700 FT-IR spectrometer.
To determine the existence states of different cobalt loadings on the carrier SBA-15, XRD measurements were carried out on the catalysts with different cobalt loadings; the results are shown in figure 3. The broad peak at 2θ = 20°–30° of SBA-15 can be attributed to the characteristic peak of SiO2 (JCPSD PDF 38-0197). After loading with cobalt oxides, the peak becomes weaker but the position does not change. This indicates that the introduction of the active component does not change the two-dimensional (2-D) ordered mesoporous structure of SBA-15, while SiO2 gradually becomes amorphous [30]. Besides, the Co3O4 diffraction peak at 2θ = 36.8° can be clearly observed in Co/SBA-15 catalysts with different cobalt loadings, and the major crystallographic plane is the (311) crystallographic plane. The (111), (220), (400), (511), and (440) crystal planes corresponding to Co3O4 crystals were also observed at 19.0°, 31.3°, 44.8, 59.3°, and 65.2° with the increase in cobalt loading (JCPDS No. 74-2120) [31]. The XRD patterns of each catalyst have distinctive characteristic peaks of spinel-structure Co3O4, indicating that the cobalt oxide exists as Co3O4 on the SBA-15 carrier.
From the XRD results, it can be observed that the cobalt loaded on SBA-15 mainly exists in the Co3O4 state. To explore the distribution of the active components on the surface of SBA-15, catalysts with different cobalt loadings were characterized by SEM, as illustrated in figure 4. In the absence of cobalt loading, the surface of SBA-15 exhibits a long rod-like loose structure with a completely smooth surface. In contrast, upon loading with various amounts of cobalt, Co3O4 particles appear on the SBA-15 surface, and their size increases with higher cobalt loading. The 20% cobalt loading leads to the sintering phenomenon of the active component [32], which is expressed as the agglomeration of Co3O4 on the surface of SBA-15. This agglomeration of the active component Co3O4 negatively impacts its catalytic effectiveness.
XPS analysis was used to determine the chemical valence distribution of cobalt in Co/SBA-15 catalysts with varying cobalt loadings. These results are presented in figure 5. The Co 2p spectrum of the Co3O4 catalyst exhibited two main peaks at Co 2p3/2 and Co 2p1/2 orbitals, as well as two satellite peaks, which is a characteristic feature of Co3O4. The two main peaks were each divided into two peaks. The main peak at Co 2p3/2 was split into two peaks at 779.82 eV and 781.16 eV, which are attributed to Co3+ and Co2+ species, respectively. Similarly, the main peak at Co 2p1/2 was divided into two peaks at 794.87 eV and 796.61 eV, which are attributed to Co3+ and Co2+ species, respectively [33]. The molar ratios of Co3+ and Co2+ contents on different catalyst surfaces were calculated by integrating the fitted curves and determining the ratio of their areas. The results are labeled in figure 5, which shows that the Co3+/Co2+ ratio was as follows: 15 wt% Co/SBA-15 (1.18) > 10 wt% Co/SBA-15 (1.05) > 20 wt% Co/SBA-15 (0.88) > 5 wt% Co/SBA-15 (0.84). Research has shown that increasing the ratio of Co3+/Co2+ promotes the formation of more active surface species, which contributes to the formation of more oxygen vacancies, thereby increasing the catalytic performance of the catalyst [34]. Therefore, the 15 wt% Co/SBA-15 catalyst has superior catalytic activity compared to other catalysts.
The degradation of toluene by Co/SBA-15 catalysts in the DBD-TCC system for different cobalt loadings is shown in figure 6. The results show that there is no significant change in toluene degradation efficiency when SBA-15 is introduced into the DBD-TCC system compared to the DBD system alone, indicating that SBA-15 as a standalone catalyst carrier has minimal catalytic effect. However, compared to the DBD system, the toluene degradation efficiency was significantly improved by the addition of the Co/SBA-15 catalyst. The toluene degradation efficiency of Co/SBA-15 catalysts with different cobalt loadings in the DBD-TCC system at a discharge voltage of 11.6 kV was as follows: 15 wt% Co/SBA-15 > 10 wt% Co/SBA-15 > 20 wt% Co/SBA-15 > 5 wt% Co/SBA-15 > SBA-15. With 15 wt% cobalt doping, Co/SBA-15 showed the highest catalytic activity with toluene degradation efficiency and CO2 selectivity of 79.5% and 82.8%, which were 13.1% and 23.3% higher than those of the DBD system alone, respectively. However, with a further increase in cobalt loading to 20 wt%, the degradation efficiency of toluene and the CO2 selectivity dropped to 73.8% and 77.8%, respectively. Consequently, based on these results, subsequent experiments will be conducted using the optimally performing 15 wt% Co/SBA-15 catalyst.
Catalyst space velocity refers to the amount of gas processed by a unit volume of catalyst within a specified time under certain conditions [35]. In this study, the gas flow rate and the radius of the filling tube were kept constant, while the catalyst dosages were adjusted to vary the catalyst space velocity in the reaction system. The corresponding space velocities are 147329 h−1, 220994 h−1, and 441989 h−1 for different catalyst dosages of 0.3 g, 0.2 g, and 0.1 g, respectively. Figure 7 illustrates the effect of the Co/SBA-15 catalyst space velocity on toluene degradation and catalyst zone temperature in the DBD-TCC system. In figure 7(a), it can be seen that as the space velocity increases, the effectiveness of the toluene degradation initially rises and then declines. The space velocity increases from 147329 h−1 to 220994 h−1, resulting in an increase in toluene degradation efficiency and CO2 selectivity from 74.8% and 80% to 81% and 84.4%, respectively. However, as the space velocity further increases from 220994 h−1 to 441989 h−1, the toluene degradation efficiency and CO2 selectivity rate decrease to 72.1% and 72.4%, respectively. The optimal space velocity that can be obtained is 220994 h−1. In figure 7(b), it is observed that as the catalyst space velocity increases, the stable temperatures of the catalyst zone are 172 °C, 219 °C, and 240 °C at space velocities of 147329 h−1, 220994 h−1, and 441989 h−1, respectively, under a discharge voltage of 11.6 kV.
Figure 8(a) illustrates the temperature variation within the catalyst zone under different voltages in the DBD-TCC system. As the discharge time increases, the temperature in the catalyst zone gradually rises and approaches stability after 20 min. The temperature stabilizes due to thermal equilibrium in the AC-DBD reactor [36]. Simultaneously, as the discharge voltage increases from 9.2 kV to 12.4 kV, the temperature correspondingly rises from 97 °C to 261 °C at 20 min. As shown in figure S2, the discharge power displays a similar trend to the temperature, initially increasing and then stabilizing, due to the absolute impedance gradually reaching stability over time [37]. In figure 8(b), the ozone concentration at the outlet after plasma treatment alone is presented. The results reveal that the ozone concentration at the outlet of the plasma region consistently remained below 10 ppm throughout the discharge process, attributed to ozone decomposition at high temperatures [38]. Therefore, almost no ozone was involved in the catalytic oxidation of toluene.
Figures 8(c) and (d) depict the influence of voltage on toluene degradation among the three different systems (DBD, DBD-TUC, and DBD-TCC). As the applied voltage increased, both the toluene degradation efficiency and the CO2 selectivity increased due to the enhanced kinetic energy and density of electrons [39], but the corresponding energy efficiency concerning toluene degradation decreased in all three systems. In addition, there is little difference in both toluene degradation efficiency and CO2 selectivity that can be observed between the DBD-TUC and DBD systems because the Co/SBA-15 catalyst cannot be activated by either heat or ozone in the DBD-TUC system. In the DBD-TCC system, significant improvements in both toluene degradation efficiency and CO2 selectivity can be obtained compared to the DBD system when the applied voltage is higher than 9.2 kV. In particular, this improvement trend is more obvious under high-voltage conditions. At the applied voltages of 10 kV, 10.8 kV, 11.6 kV, and 12.4 kV, the toluene degradation efficiencies increased by 5.1%, 12.3%, 14.1%, and 17%, respectively, compared to the DBD system, while the corresponding CO2 selectivity increased by 8.7%, 11.6%, 17.4%, and 21.2%. Additionally, at 12.4 kV, the energy efficiency of the DBD-TCC system increased by 27% compared to the DBD system. The results indicate that the Co/SBA-15 catalyst can be activated when the AC-DBD voltage exceeds 10 kV, generating thermal effects that raise the temperature of the catalyst zone to above 126 °C.
To confirm the temperature contribution to activation of the Co/SBA-15 catalyst and the corresponding toluene decomposition, thermal catalytic oxidation experiments were performed using the same DBD-TCC reactor without discharge. The experimental results are shown in figure 9. It can be observed that at a temperature of 97 °C, the catalyst exhibits minimal toluene degradation performance (2.3%) while, as the temperature increases above 126 °C, the catalyst is gradually activated and the toluene degradation effects gradually increase. At 261 °C, the toluene degradation efficiency reaches 55.7%. This trend aligns with the activation trend of the Co/SBA-15 catalyst by the thermal effects generated by AC-DBD in the DBD-TCC system when the voltage exceeds 10 kV (resulting in a temperature exceeding 126 °C). However, the toluene degradation efficiency of the thermal catalytic oxidation using the Co/SBA-15 catalyst is still lower compared to the DBD-TCC system, confirming the effectiveness of the DBD-TCC system.
As the plasma catalytic degradation of VOCs continues, the organic by-products generated from the incomplete oxidation of VOCs cover the active sites on the catalyst surface, leading to a decrease in catalytic activity and affecting the degradation effects [40]. Figure S3 demonstrates that the Co/SBA-15 catalyst maintains high stability in the DBD-TCC system. To investigate the chemical bonds in both fresh and used Co/SBA-15 catalysts, the FT-IR spectrum was analyzed and the results are shown in figure 10(a). The FT-IR spectra of the Co/SBA-15 catalyst before and after use exhibit minimal differences. The absorption peaks observed at 464 cm−1, 807 cm−1, and 1089 cm−1 of Co/SBA-15 are attributed to the bending vibrational peaks, telescopic vibrational peaks, and antisymmetric telescopic symmetry peaks of the Si–O–Si bond in the SBA-15 carrier [41]. Additionally, the peaks at 1637 and 3448 cm−1 are associated with the bending vibrations of hydroxyl or silanol groups (Si–OH) in the adsorbed water molecules [42]. In comparison with the fresh samples, the used Co/SBA-15 catalyst exhibits a distinct peak at 2930 cm−1, possibly attributed to the –CH2– asymmetric stretching peaks. This observation may be due to the adsorption of organic substances on the catalyst surface. In section 3.2, the role of cobalt in the enhancement of toluene degradation performance in the Co/SBA-15 catalyst was demonstrated. Here, XPS analysis was used to examine the changes in the chemical state of the catalyst surface. Figure 10(b) shows the Co 2p spectra of the catalysts. The results indicate a decrease in the ratio of Co3+/Co2+ on the used catalysts from 1.16 to 0.97 compared to the fresh catalysts.
The gas phase products of DBD and DBD-TCC degradation were identified by GC-MS, as shown in table 1. It can be observed that following the DBD stage, toluene degradation was not complete, with most of the intermediate products still present in the form of benzene rings. However, after passing through the catalytic region of the DBD-TCC system, a higher yield of ring-opening products was generated, indicating the advancement of toluene conversion towards final products.
Name | Retention time (min) | Chemical structure | DBD | DBD-TCC |
Benzene | 3.31 | ![]() |
√ | √ |
Trioxane | 3.72 | ![]() |
√ | |
Acetic anhydride | 4.49 | ![]() |
√ | |
2-Butanone | 7.96 | ![]() |
√ | |
1,4-Xylene | 8.38 | ![]() |
√ | |
2-Cyclopentene-1,4-dione | 8.81 | ![]() |
√ | |
Itaconic anhydride | 10.69 | ![]() |
√ | √ |
Benzyl alcohol | 13.78 | ![]() |
√ | √ |
1-Nonanal | 15.87 | ![]() |
√ | |
Nitrobenzene | 15.42 | ![]() |
√ | |
2-Nitrophenol | 16.43 | ![]() |
√ | √ |
Hydroxylamine, O-decyl- | 18.72 | ![]() |
√ |
The thermal effect generated by a high-frequency AC-DBD plasma discharge process results in energy waste. To address this, we designed the DBD-TCC reactor to investigate the potential utilization of this wasted heat for the activation of Co/SBA-15 catalysts in the degradation of VOCs. Initially, we observed that the degradation effect of toluene exhibited an increase followed by a subsequent drop as the cobalt loading in the catalyst was increased. This is because the number of active sites in a catalyst tends to increase with higher cobalt doping [43]. Nevertheless, excessive cobalt doping can potentially cause the aggregation of active species and harm the mesoporous structure, leading to alterations in the physicochemical properties and subsequent suppression of catalytic activity [44].
Furthermore, we found that in the DBD-TCC system, the degradation efficiency of toluene initially increased and then decreased with increasing catalyst space velocity. As the catalyst space velocity is low, the residence time of reactant molecules in the catalyst region is prolonged [45, 46]. Consequently, the degradation efficiency of toluene is expected to decrease with a higher catalyst space velocity. However, in the DBD-TCC system, there was a deviation from this expected trend. The reason for this can be attributed to the increase in the space velocity, which led to an increase in the temperature of the catalyst zone. An increase in temperature can enhance lattice oxygen activation [47] while, simultaneously, the catalyst provides more active sites to participate in the reaction, thus improving the degradation efficiency of toluene. However, when the space velocity is further increased, although the temperature of the catalyst zone increases, the number of active sites provided by the catalyst is limited. Therefore, reactant molecules cannot react sufficiently with the catalyst, leading to a decrease in toluene efficiency. This indirectly indicates that temperature plays a predominant role in the activation of the Co/SBA-15 catalyst in the DBD-TCC system.
We have demonstrated that in the DBD-TCC system, the catalyst region can be heated by the AC-DBD plasma to a sufficiently high temperature to satisfy the catalytic activation temperature requirements. However, since the DBD region is separated from the catalyst region, the catalyst fails to exhibit catalytic and toluene degradation effects. This is because the reactivity of catalytic oxidation is closely related to temperature. Increasing the catalyst temperature can enhance the availability of reactive oxygen species on the catalyst surface, thereby improving the toluene degradation effects [48]. Additionally, we verified that the heat wasted during the high-frequency AC-DBD process can be utilized to heat the catalyst.
Moreover, our findings indicated that the Co/SBA-15 catalyst exhibited stability to degrade toluene inside the DBD-TCC system and the active centers and surface structure of the catalyst remained stable with no evident signs of poisoning. The slight decrease in Co3+/Co2+ after the reaction is attributed to the reduction of Co3+ to Co2+ during the catalytic oxidation process of toluene to the intermediate products, CO2, and H2O, following the Mars−van Krevelen (MvK) mechanism [49]. However, the ratio of Co3+/Co2+ after the reaction compared to before the reaction did not show a significant decrease, indicating that its active site can maintain stability after undergoing a cyclic reaction [50].
The toluene decomposition process in the DBD-TCC system involves plasma treatment followed by catalytic oxidation over the Co/SBA-15 catalyst. Figure 11 illustrates the degradation mechanisms involved in the DBD-TCC system removal of toluene. In the DBD process, high-energy electrons generated by the plasma play a crucial role in the stepwise decomposition, as well as oxidation of toluene and intermediates in combination with active species generated in the background gases, such as O and OH radicals, and excited N2 [51, 52]. Furthermore, these high-energy electrons can directly collide with toluene molecules, transforming them into smaller molecular substances [53]. The extraction of hydrogen from the methyl group can result in the formation of benzyl. Additionally, the excitation of the C–C bond between the methyl group and the benzene ring induces nitrogen and electron cleavage, leading to the generation of phenyl radicals and methyl radicals. Benzyl can react with OH radicals to form benzyl alcohol, and phenyl can react with CH3, H, NO2, and O radicals to form 1,4-Xylene, Benzene, Nitrobenzene, and 2-Cyclopentene-1,4-dione, respectively [54]. Next, aromatic intermediates collide with energetic electrons and reactive species, resulting in the rupture of aromatic rings and stepwise oxidation into CO2 and H2O [55]. In the catalytic oxidation process within the DBD-TCC system, the Co/SBA-15 catalyst can be activated by the heat generated from the high-frequency AC-DBD. This activation enables further treatment of untreated toluene and the intermediate products produced by DBD. The MvK mechanism, widely accepted in transition metal oxides, is generally considered to dominate the reaction pathway in this step [56, 57]. According to the different positions on the catalyst, whole lattice oxygen can be divided into surface lattice oxygen and lattice oxygen (Olatt) [47]. Since the reaction occurs on the catalyst surface, surface lattice oxygen directly participates in the reaction, while gaseous oxygen can be replenished through surface oxygen vacancies to compensate for consumed oxygen species [58]. Initially, toluene and intermediates are adsorbed onto the catalyst surface and oxidized by Olatt, while Co3+ sites are reduced to Co2+, and oxygen vacancies are formed in situ. Subsequently, the oxygen vacancies can be replenished by O2 in the gas phase, leading to the reactivation of adsorbed oxygen and the re-oxidation of Co2+ to Co3+. The intermediates generated in the plasma region during this process are converted to 2-Butanone, 1-Nitrophenol, Acetic anhydride, and Trioxane, eventually mineralized to CO2 and H2O together with undegraded toluene.
In this study, an innovative DBD-TCC system was constructed by utilizing high-frequency AC-DBD and its heat-induced activation of the Co/SBA-15 catalyst for toluene treatment. The characterization and performance testing results of Co/SBA-15 catalysts with different loadings indicate that the 15 wt% Co/SBA-15 catalyst had the highest Co3+/Co2+ and demonstrated the best toluene degradation performance. In addition, when the catalyst space velocity is 220994 h−1, it exhibits optimal toluene degradation performance in the DBD-TCC system, possibly due to its suitable number of active sites and the catalytic zone being heated to a sufficiently high temperature (219 °C). The improved toluene degradation and energy efficiency of the DBD-TCC system compared to the DBD and DBD-TUC systems confirm the crucial role of plasma thermal effects in the activation of the Co/SBA-15 catalyst. At discharge voltages exceeding 10 kV, the heat generated by AC-DBD can elevate the temperature of the Co/SBA-15 catalyst zone above 126 °C. The results of thermal catalytic oxidation experiments illustrate that the temperature response trend of the Co/SBA-15 catalyst aligns with that in the DBD-TCC system. Characterization of the Co/SBA-15 catalyst before and after reaction in the DBD-TCC system reveals that it retains its activity and structural integrity, confirming its effectiveness in the DBD-TCC system. The pathway of toluene degradation in the DBD-TCC system indicates that toluene is initially partially decomposed into intermediate products within the plasma region. Then, these intermediate products migrate to the surface of the Co/SBA-15 catalyst, which undergoes thermal activation due to AC-DBD, along with any unreacted toluene. Via the MvK mechanism, toluene undergoes deep oxidation, ultimately converting into CO2 and H2O. This work establishes a novel system for employing plasma-generated heat in catalytic oxidation without the need for additional heating, providing a new approach to reduce energy waste and enhance energy efficiency in AC-DBD plasma treatment of VOCs.
This work was supported by National Natural Science Foundation of China (No. 52177130) and the Key Projects for Industrial Prospects and Core Technology Research in Suzhou City (No. SYC2022029).
[1] |
He C et al 2019 Chem. Rev. 119 4471 doi: 10.1021/acs.chemrev.8b00408
|
[2] |
Guo Y L et al 2021 Appl. Catal. B: Environ. 281 119447 doi: 10.1016/j.apcatb.2020.119447
|
[3] |
Batault F et al 2015 Chem. Eng. J. 264 197 doi: 10.1016/j.cej.2014.10.089
|
[4] |
Dumont E et al 2011 Chem. Eng. J. 168 241 doi: 10.1016/j.cej.2010.12.073
|
[5] |
Mao G Z et al 2022 J. Membr. Sci. 652 120443 doi: 10.1016/j.memsci.2022.120443
|
[6] |
Yang X Q et al 2020 Appl. Catal. B: Environ. 263 118355 doi: 10.1016/j.apcatb.2019.118355
|
[7] |
Li S J et al 2020 Chem. Eng. J. 388 124275 doi: 10.1016/j.cej.2020.124275
|
[8] |
Xie W et al 2024 J. Hazard. Mater. 461 132518 doi: 10.1016/j.jhazmat.2023.132518
|
[9] |
Jin M Y et al 2023 Fuel 342 127921 doi: 10.1016/j.fuel.2023.127921
|
[10] |
Zhang Z X et al 2016 Catal. Today 264 270 doi: 10.1016/j.cattod.2015.10.040
|
[11] |
Lu S Y et al 2012 IEEE Trans. Plasma Sci. 40 2151 doi: 10.1109/TPS.2012.2206119
|
[12] |
Lu B et al 2008 J. Mater. Eng. Perform. 17 428 doi: 10.1007/s11665-007-9157-5
|
[13] |
Mustafa M F et al 2018 J. Hazard. Mater. 347 317 doi: 10.1016/j.jhazmat.2018.01.021
|
[14] |
Ragazzi M et al 2014 Waste Manag. 34 2400 doi: 10.1016/j.wasman.2014.07.026
|
[15] |
Delagrange S, Pinard L and Tatibouët J M 2006 Appl. Catal. B: Environ. 68 92 doi: 10.1016/j.apcatb.2006.07.002
|
[16] |
Rodrigues F, Pascoa J and Trancossi M 2018 Exp. Therm. Fluid Sci. 90 55 doi: 10.1016/j.expthermflusci.2017.09.005
|
[17] |
Nguyen D B and Lee W G 2014 J. Ind. Eng. Chem. 20 972 doi: 10.1016/j.jiec.2013.06.031
|
[18] |
Wang Q et al 2018 Plasma Sci. Technol. 20 035404 doi: 10.1088/2058-6272/aaa357
|
[19] |
Li M Y et al 2021 J. Environ. Chem. Eng. 9 105529 doi: 10.1016/j.jece.2021.105529
|
[20] |
Wang B F et al 2018 Catal. Surv. Asia 22 73 doi: 10.1007/s10563-018-9241-x
|
[21] |
Ren Y W et al 2023 ACS Catal. 13 8293 doi: 10.1021/acscatal.3c01036
|
[22] |
Ojala S et al 2011 Appl. Catal. B: Environ. 108–109 22 doi: 10.1016/j.apcatb.2011.07.031
|
[23] |
Wu P et al 2021 Environ. Sci. Technol. 55 4268 doi: 10.1021/acs.est.0c08179
|
[24] |
Lyu Y et al 2020 Environ. Sci. Pollut. Res. 27 2482 doi: 10.1007/s11356-019-07037-2
|
[25] |
Solsona B et al 2008 Appl. Catal. B: Environ. 84 176 doi: 10.1016/j.apcatb.2008.03.021
|
[26] |
Kołodziej A et al 2012 Chem. Eng. J. 200–202 329 doi: 10.1016/j.cej.2012.06.067
|
[27] |
Ren Q M et al 2019 Catal. Today 332 160 doi: 10.1016/j.cattod.2018.06.053
|
[28] |
Bai G M et al 2013 Appl. Catal. A: Gen. 450 42 doi: 10.1016/j.apcata.2012.09.054
|
[29] |
Wen M et al 2021 Microporous Mesoporous Mater. 322 111156 doi: 10.1016/j.micromeso.2021.111156
|
[30] |
Verma P et al 2020 Nanoscale 12 11333 doi: 10.1039/D0NR00732C
|
[31] |
Miao C et al 2020 New J. Chem. 44 11557 doi: 10.1039/D0NJ00091D
|
[32] |
Ronduda H et al 2022 RSC Adv. 12 33876 doi: 10.1039/D2RA06053A
|
[33] |
Wang K et al 2017 ACS Appl. Mater. Interfaces 9 16128 doi: 10.1021/acsami.7b01142
|
[34] |
Zhao Q et al 2020 Chemosphere 244 125532 doi: 10.1016/j.chemosphere.2019.125532
|
[35] |
Wu Z M et al 2015 Mater. Res. Bull. 70 567 doi: 10.1016/j.materresbull.2015.05.028
|
[36] |
Dinh M T N et al 2016 J. Hazard. Mater. 314 88 doi: 10.1016/j.jhazmat.2016.04.027
|
[37] |
Nguyen D B et al 2020 Int. J. Hydrogen Energy 45 18519 doi: 10.1016/j.ijhydene.2019.06.167
|
[38] |
Itoh H, Taguchi M and Suzuki S 2020 J. Phys. D: Appl. Phys. 53 185206 doi: 10.1088/1361-6463/ab71a9
|
[39] |
Zhao Q J et al 2022 Appl. Therm. Eng. 217 119276 doi: 10.1016/j.applthermaleng.2022.119276
|
[40] |
Jin Q et al 2016 Chem. Eng. J. 286 300 doi: 10.1016/j.cej.2015.10.070
|
[41] |
Xu S Q et al 2019 Ind. Eng. Chem. Res. 58 13013 doi: 10.1021/acs.iecr.9b01821
|
[42] |
Zhang B J et al 2022 Ind. Eng. Chem. Res. 61 1044 doi: 10.1021/acs.iecr.1c02504
|
[43] |
Xu X X et al 2017 Catal. Today 281 527 doi: 10.1016/j.cattod.2016.03.036
|
[44] |
Einaga H and Futamura S 2004 J. Catal. 227 304 doi: 10.1016/j.jcat.2004.07.029
|
[45] |
Zhu Y C et al 2024 Sep. Purif. Technol. 328 124993 doi: 10.1016/j.seppur.2023.124993
|
[46] |
Jiang H B et al 2020 RSC Adv. 10 5116 doi: 10.1039/c9ra10601d
|
[47] |
Bo Z et al 2020 ACS Catal. 10 4420 doi: 10.1021/acscatal.9b04844
|
[48] |
Ren Y W et al 2024 ACS Catal. 14 4340 doi: 10.1021/acscatal.3c06237
|
[49] |
Zhong J P et al 2020 Chem. Eng. J. 397 125375 doi: 10.1016/j.cej.2020.125375
|
[50] |
Alex C et al 2020 ACS Appl. Energy Mater. 3 5439 doi: 10.1021/acsaem.0c00297
|
[51] |
Zhu X B et al 2015 Appl. Catal. B: Environ. 170–171 293 doi: 10.1016/j.apcatb.2015.01.032
|
[52] |
Chang T et al 2018 Chem. Eng. J. 348 15 doi: 10.1016/j.cej.2018.04.186
|
[53] |
Dobslaw D et al 2017 J. Environ. Chem. Eng. 5 5501 doi: 10.1016/j.jece.2017.10.015
|
[54] |
Huang H B et al 2011 J. Mol. Catal. A: Chem. 336 87 doi: 10.1016/j.molcata.2011.01.002
|
[55] |
Yi H H et al 2017 Energy Fuels 31 11217 doi: 10.1021/acs.energyfuels.7b01657
|
[56] |
Xie S H et al 2017 J. Catal. 352 282 doi: 10.1016/j.jcat.2017.05.016
|
[57] |
Dong Y L et al 2020 Chem. Eng. J. 388 124244 doi: 10.1016/j.cej.2020.124244
|
[58] |
Qin Y et al 2019 J. Catal. 380 21 doi: 10.1016/j.jcat.2019.09.027
|
[1] | Kun YANG, Hongwei SHEN, Yueyue LIU, Yang LIU, Pingji GE, Dezheng YANG. Degradation of tiamulin by a packed bed dielectric barrier plasma combined with TiO2 catalyst[J]. Plasma Science and Technology, 2022, 24(9): 095504. DOI: 10.1088/2058-6272/ac6d41 |
[2] | Baowei WANG (王保伟), Chao WANG (王超), Shumei YAO (姚淑美), Yeping PENG (彭叶平), Yan XU (徐艳). Plasma-catalytic degradation of tetracycline hydrochloride over Mn/γ-Al2O3 catalysts in a dielectric barrier discharge reactor[J]. Plasma Science and Technology, 2019, 21(6): 65503-065503. DOI: 10.1088/2058-6272/ab079c |
[3] | Jingyu REN (任景俞), Nan JIANG (姜楠), Kefeng SHANG (商克峰), Na LU (鲁娜), Jie LI (李杰), Yan WU (吴彦). Evaluation of trans-ferulic acid degradation by dielectric barrier discharge plasma combined with ozone in wastewater with different water quality conditions[J]. Plasma Science and Technology, 2019, 21(2): 25501-025501. DOI: 10.1088/2058-6272/aaef65 |
[4] | Lin WANG (王林), Junkang YAO (姚军康), Zheng WANG (王政), Hongqiao JIAO (焦洪桥), Jing QI (齐静), Xiaojing YONG (雍晓静), Dianhua LIU (刘殿华). Fast and low-temperature elimination of organic templates from SBA-15 using dielectric barrier discharge plasma[J]. Plasma Science and Technology, 2018, 20(10): 101001. DOI: 10.1088/2058-6272/aad547 |
[5] | Shoufeng TANG (唐首锋), Na LI (李娜), Jinbang QI (綦金榜), Deling YUAN (袁德玲), Jie LI (李杰). Degradation of phenol using a combination of granular activated carbon adsorption and bipolar pulse dielectric barrier discharge plasma regeneration[J]. Plasma Science and Technology, 2018, 20(5): 54013-054013. DOI: 10.1088/2058-6272/aaa7e9 |
[6] | Xu CAO (曹栩), Weixuan ZHAO (赵玮璇), Renxi ZHANG (张仁熙), Huiqi HOU (侯惠奇), Shanping CHEN (陈善平), Ruina ZHANG (张瑞娜). Conversion of NO with a catalytic packed-bed dielectric barrier discharge reactor[J]. Plasma Science and Technology, 2017, 19(11): 115504. DOI: 10.1088/2058-6272/aa7ced |
[7] | DI Lanbo (底兰波), ZHAN Zhibin (詹志彬), ZHANG Xiuling (张秀玲), QI Bin (亓滨), XU Weijie (徐伟杰). Atmospheric-Pressure DBD Cold Plasma for Preparation of High Active Au/P25 Catalysts for Low-Temperature CO Oxidation[J]. Plasma Science and Technology, 2016, 18(5): 544-548. DOI: 10.1088/1009-0630/18/5/17 |
[8] | WANG Jingting (王婧婷), CAO Xu (曹栩), ZHANG Renxi (张仁熙), GONG Ting (龚挺), HOU Huiqi (侯惠奇), CHEN Shanping (陈善平), ZHANG Ruina (张瑞娜). Effect of Water Vapor on Toluene Removal in Catalysis-DBD Plasma Reactors[J]. Plasma Science and Technology, 2016, 18(4): 370-375. DOI: 10.1088/1009-0630/18/4/07 |
[9] | FU Tingjun(付廷俊), HUANG Chengdu(黄承都), LV Jing(吕静), LI Zhenhua(李振花). Fischer-Tropsch Performance of an SiO 2 -Supported Co-Based Catalyst Prepared by Hydrogen Dielectric-Barrier Discharge Plasma[J]. Plasma Science and Technology, 2014, 16(3): 232-238. DOI: 10.1088/1009-0630/16/3/11 |
[10] | BAI Suli(白素丽), HUANG Chengdu(黄承都), LV Jing(吕静), LI Zhenhua (李振花). Performance of Cobalt-based Fischer-Tropsch Synthesis Catalysts using Dielectric-Barrier Discharge Plasma as an Alternative to Thermal Calcination?[J]. Plasma Science and Technology, 2012, 14(1): 54-57. DOI: 10.1088/1009-0630/14/1/12 |
Name | Retention time (min) | Chemical structure | DBD | DBD-TCC |
Benzene | 3.31 | ![]() |
√ | √ |
Trioxane | 3.72 | ![]() |
√ | |
Acetic anhydride | 4.49 | ![]() |
√ | |
2-Butanone | 7.96 | ![]() |
√ | |
1,4-Xylene | 8.38 | ![]() |
√ | |
2-Cyclopentene-1,4-dione | 8.81 | ![]() |
√ | |
Itaconic anhydride | 10.69 | ![]() |
√ | √ |
Benzyl alcohol | 13.78 | ![]() |
√ | √ |
1-Nonanal | 15.87 | ![]() |
√ | |
Nitrobenzene | 15.42 | ![]() |
√ | |
2-Nitrophenol | 16.43 | ![]() |
√ | √ |
Hydroxylamine, O-decyl- | 18.72 | ![]() |
√ |