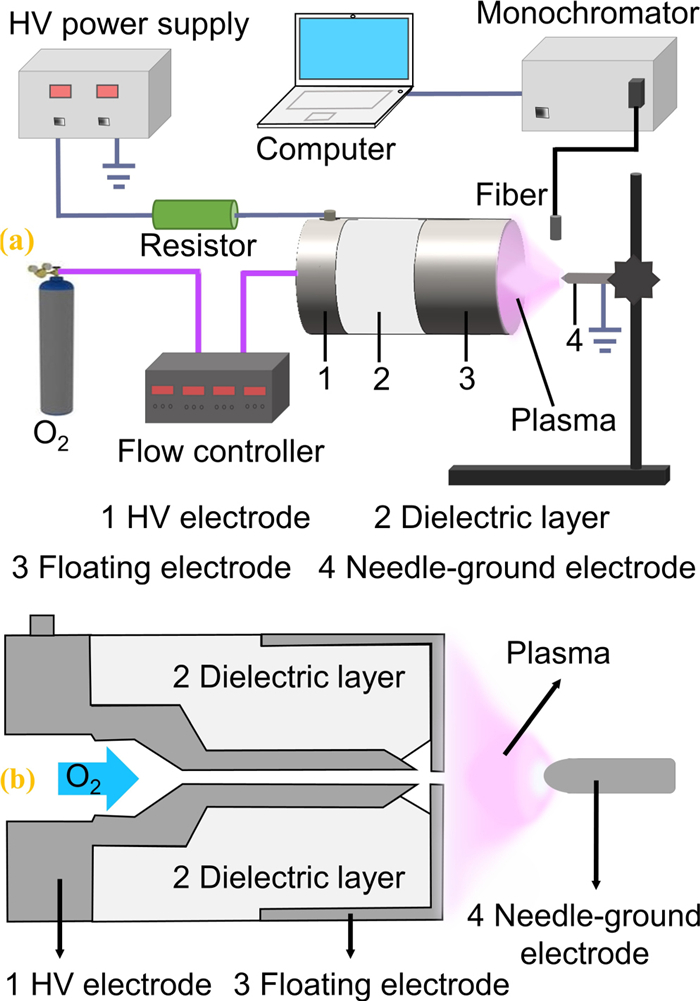
Citation: | Min ZHANG, Yunhu LIU, Yao LI, Shuqi LI, Hao YUAN, Jianping LIANG, Xiongfeng ZHOU, Dezheng YANG. Generation of atmospheric pressure air diffuse discharge plasma in oxygen enriched working gas with floating electrode[J]. Plasma Science and Technology, 2023, 25(4): 045405. DOI: 10.1088/2058-6272/aca5f3 |
In this work, a floating electrode is employed to generate a stable large-area diffuse discharge plasma under an open oxygen-rich environment. The discharge image and the optical emission spectra of the N2(C-B),
Non-thermal plasma, which is known as high electron temperature and low gas temperature, is widely used in biomedicine [1–3], food processing [4–6], material surface modification [7–9], chemical industry [10, 11] and other fields. Most of those applications rely on reactive oxygen species (ROS) and require uniform discharge (e.g. glow). Producing a high density of ROS (e.g. OH, O), which can participate in the reactions with the target material, is of great value [12, 13]. The atmospheric pressure discharge (especially in the bare electrode structure) is easily transformed into the filament, spark discharge, or arc discharge [14–17], which will damage the sample and limit the application who is sensitive to discharge uniformity. Therefore, obtaining a diffuse or uniform discharge in atmospheric air is a challenge attracting countless scientists.
The key to obtain a uniform or diffuse discharge is to prevent the transition from glow or uniform to the filament, spark discharge, arc discharge, etc. In recent years, researchers have used the forms of designing excitation power supply, adjusting discharge parameters and optimizing electrode structure to generate diffuse discharge plasma. They have carried out a large number of experimental and theoretical studies. Zhang et al demonstrated that a smaller pulse peak voltage or discharge gap is more conducive to improving the discharge uniformity of nanosecond-pulse discharge [18]. The studies of Shi et al show that compared with naked electrodes, the dielectric insulation of electrodes is conducive to the stability of radio-frequency glow, and the reason is that the dielectric barrier can prevent the infinite growth of discharge current and thus reduce the tendency of the glow-to-spark transition (GST) [19–21]. In addition, GST can also be regulated by specially designed electrode structures. Kanazawa et al proposed to use different grounding electrodes and nozzle shapes to control the DC corona discharge mode in atmospheric air [22].
To generate diffuse discharge, various floating electrodes (FEs) which are isolated (voltage-free, ungrounded) conductors are added to the electrode configuration [23, 24]. The FE added between the high-voltage electrode and the ground electrode will form an equipotential body, and the consequential change of the original electric field distribution affects the discharge operation. The preferred electric field can be obtained by changing the shape and position of FE. In recent years, the FE structure has been used to stabilize the discharge plasma and increase the length of the plasma feather, so as to improve the performance of the plasma source. Deepak et al show that the ring or helix FE is beneficial to restrict the transition of glow to arc and effectively increase the length of the plasma jet [25, 26]. A plasma reactor with a plate FE, which has a solid metal top half and a metallic foam bottom half, designed by Zhu et al, increases the volume of plasma light-emitting area and improves the efficiency of hydrogen production [27].
In this paper, in order to overcome the discharge instability under the bare electrode structure, we developed a cap FE configuration to generate a stable diffuse discharge in a large electrode gap using oxygen enriched working gas under the excitation of a DC power supply. The formation mechanism of the diffuse discharge plasma with FE is analyzed, and the effects of FE on breakdown voltage and GST voltage are studied. In addition, emission spectra of the active particles and discharge images are recorded to discuss the discharge characteristics, active species, and gas temperature.
The experimental setup is shown in figure 1(a) and the electrode structure of the plasma reactor is detailed in figure 1(b). The experimental setup is composed of a DC power supply, a discharge reactor, a gas inlet system and an optical detection system. The DC power supply can provide a voltage range of 0–60 kV and this work uses negative polarity DC. A 3 kΩ ballast resistor is connected in series between the DC power supply and the high-voltage (HV) electrode to stabilize the discharge current. The discharge reactor consists of an HV electrode, a Teflon dielectric layer, an FE and a needle-ground electrode. The sectional view of the reactor is shown in figure 1(b). All of three electrode materials are 304 stainless steel. The inner diameter of the nozzles for both the HV electrode and the FE is 1.5 mm, and the outer diameter of the FE is 30 mm. The thickness of FE is assumed to be 1 mm, and the distance between the nozzle of the HV electrode and the circular hole of the central axis of the FE is 2 mm. The distance between the FE and the needle-ground electrode which is defined as the electrode gap is adjusted in the range of 10–30 mm. In other words, compared with using FE, the electrode gap without FE (the distance between the HV electrode and the needle-ground electrode) is 3 mm larger. In the plasma reactor, the gas inlet is connected with the HV electrode, where high-purity oxygen (99.999%) is injected into the reactor as the plasma working gas, and the gas flow rate, controlled by mass flow controllers, is kept at 0.7 l min-1 to generate an oxygen-enriched discharge plasma. An optical fiber collects the photons radiating from the discharge area and transports them to a grating monochromator (Andor SR-750i, the grating groove is 2400 lines mm-1 and glancing wavelength is 300 nm) in the optical detection system. A CCD camera (Andor Newton DU 940P-BV) is equipped with the grating monochromator for photoelectric conversion and analog signal to digital signal conversion. Finally, the corresponding optical emission spectra (OES) are displayed on a computer. In this paper, the observation zone of all the spectral data collected in the experiment is always located at a position 2 mm away from the tip of the needle-ground electrode in the horizontal axis of the plasma reactor. Moreover, the spectra are measured three times in each experimental condition, and the emission spectra intensities, the vibrational temperature, and the standard deviations are obtained by calculating the rotational temperature separately.
In order to clarify the effect of the FE on the stability of the discharge, the applied voltage is measured when the discharge starts and the discharge form transfers to spark with and without the FE. We define the above two voltages as breakdown voltage and GST voltage, respectively. Figure 2 shows the GST voltage and breakdown voltage of the discharge varying as function of the length of electrode gap under the gas flow rate of 0.7 l min-1. In the measurement, the location of HV electrode and FE is fixed and the electrode gap is changed from 10 to 30 mm by moving the needle-ground electrode. It is found that both the GST voltage and the breakdown voltage show a rising tendency when electrode gap is increased. The rising tendency is mainly caused by the increase of the value pd, which makes the gas breakdown more difficult [28, 29]. The p and d represent pressure and electrode gap, respectively.
It is also exhibited clearly in figure 2 that the GST voltage and breakdown voltage are higher with the FE than those without FE under the same conditions, and it is noteworthy that compared with non-FE, the range of applied voltage to maintain corona discharge mode and diffuse discharge mode is enlarged with a FE. Especially in the electrode gap of 15 and 20 mm, the applied voltage range of stable discharge is expanded from 14–26 to 15–27.5 kV and from 15–19 to 17–28 kV, respectively. The presence of the FE changes the distribution of the electric field (see figure 4(b)) to be concentrated on the center axis of the high voltage electrode and the needle-ground electrode. In this case, the electric field (see figure 4(a)) between the FE and the needle-ground electrode is similar to the electric field of the pin-plate electrode. In other words, the electric field distributed near the FE is weak while the electric field intensity near the needle electrode is strong. Therefore, ignition or transition of diffuse to spark requires a higher applied voltage when the FE is added [30, 31]. Thus, it is able to maintain a stable discharge (corona discharge and diffuse discharge) over a relatively wide range of applied voltages while the FE is added.
Figure 3 shows the discharge images and corresponding discharge light intensity spatial resolution with FE under the applied voltages of 18 kV, 23 kV and 27 kV. The discharge images are captured by a Cannon EOS 5D Mark IV digital camera. The gas flow rate and the electrode gap are set as 0.7 l min-1 and 15 mm, respectively. The MATLAB code [32] is used to analyze the discharge light intensity of the observation area as shown in figure 3(b). It is also can be seen that near the tip of the needle-ground electrode, the discharge light intensity is the strongest under the three applied voltages. As shown in figure 3(a), it is a corona discharge at the tip of the needle-ground electrode as applied voltage is set as 18 kV. The corona gradually transits to a more uniform diffuse discharge, with the area of discharge expanding from the needle-ground electrode to the surface of the FE as the applied voltage increases from 18 to 23 kV. When the applied voltage increases from 23 to 27 kV, the volume of the diffuse discharge area increases obviously and the discharge is more uniform. Especially when the voltage is 27 kV, the plasma can be distributed to the whole bottom of the FE. In addition, the stronger discharge light is observed in the axis direction of the electrode gap, indicating that energetic electrons are more likely to appear in the axis direction of the electrode gap than those in the radial direction during the discharge process. Accordingly, the number of positive ions which are resident in the axis of electrodes is the largest.
For the sake of verifying the effect of the FE, we use COMSOL to simulate the electric intensity distribution field before breakdown to understand the initial state of the discharge. According to the actual size and material, the two-dimensional models of plasma reactor with and without FE structure are established. The mesh of the whole model is divided by refinement method. The two electrode structures are simulated by using the electrostatic field module in the software using finite element method. During the calculation, the needle-ground electrode is grounded and the potential of the HV electrode is set to the breakdown voltage (-15 kV and -14 kV) of the two structures, respectively. The spatial distribution of the potential near the FE is calculated under the same conditions.
The electric intensity distributions of diffuse discharge plasma with FE and without FE are shown in figures 4(a) and (b), respectively. The electric field intensity distribution is described in section 3.1, and in both of the electrode structures, the strongest electric field is located at the tip of the HV electrode and the needle-ground electrode. Compared with that using FE, the electric field strength near the needle-ground electrode is weaker without FE but it is easier to breakdown and discharge, which is consistent with the results shown in figure 2(b). It is because a lot of input energy is taken up by the discharge between the HV electrode and FE. As can be seen in figure 4(a), although the electric field strength at the needle-ground electrode (3.15×106 V m-1) is larger than that of HV electrode tip (1.55×106 V m-1), discharge is more likely to occur between the HV electrode and FE because of the small distance between them. According to the study of Liu et al [33], it can be speculated that there is discharge between the HV electrode and FE, but it could not be observed directly in the discharge images due to the opaque electrode material.
According to the simulation results of electric potential in figure 4(c), FE forms an equipotential body with an electric potential of -13024 V in the electric field before gas breakdown. With the increase of applied voltage, gas breakdown is easier to occur between the HV electrode and the FE due to the very small radius of curvature of the HV electrode and the relatively small gap which results in a strong electric field. The electron density increases and the plasma resistance value is very small after the breakdown in the discharge region. Accordingly, the potentials of the FE and the HV electrode are approximately equal. At this point, the HV electrode and the FE can be approximately regarded as a virtual electrode and the above discharges provide seed electrons for the discharge between the virtual electrode and the needle-ground electrode. According to the above description, the electrons emitted by the virtual electrode move towards the needle-ground electrode in the electric field. Due to the strong electric field near the nozzle of virtual electrode shown in figure 4(a), the electrons can obtain high energy in a short distance and collide with the heavy particles to stimulate ionization and thus form electron avalanche [29]. The electron avalanche moves towards the anode with a velocity while positive ions and neutral particles are seen as stationary. A positive corona is then formed at the needle-ground electrode. Under applied voltage of 18 kV, the electric field intensity is not sufficient to sustain ionization at a great distance from the cathode, thus the electron avalanche propagation channel is destroyed and the discharge area does not expand to the virtual electrode. Therefore, it is a corona discharge in 18 kV. As the voltage further increased to 23 kV, corona discharge developed into diffuse discharge by which the electrode gap is bridged as shown in figure 3(a). The diffuse discharge maintains the bridging of the discharge gap while the applied voltage increases from 23 to 27 kV. When the applied voltage increases further, the spark will be observed in the discharge region and a more detailed study has been conducted in the study of Shao et al [17].
The OES contain information such as the active species and their radiation types and the vibrational population, which is essential for the diagnosis of plasma characteristics. Figure 5 records the typical OES of the plasma in the ranges of 300–420 nm and 600–800 nm. The applied voltage, electrode gap and gas flow rate are kept at 23 kV, 15 mm and 0.7 l min-1, respectively. The OES mainly consists of the second positive bands N2(C-B), the first negative bands
The emission spectrum intensity is mainly related to the population and radiation rate of the corresponding particles and it is specifically discussed in the study of Zhao et al [34]. Among them, the relevant reactions of the main reactive nitrogen species are as follows:
e+N2(X)→N2(C)+e | (1) |
N2(C)→N2(B)+hν | (2) |
N2(C)+N2/O2→products | (3) |
e+N2(X)→N2(B)+e | (4) |
N2(B)→N2(A)+hν | (5) |
N2(B)+N2/O2→products | (6) |
e+N2(X)→N+2(B)+2e | (7) |
N+2(B)→N+2(X)+hν. | (8) |
The spectrum of N2(C-B) in figure 5(b) is the secondary diffraction spectrum. There are two main ways to produce O(3p). One way is that O2 directly collides with high-energy electrons and is ionized and excited to produce O(3p) (reactions (9)–(11)) [35]. The other way is that the O2 is ionized by the metastable N2(A) [36] and then oxygen atom in its product is excited to O(3p) as in reactions (12) and (13) [35]
e+O2→e+O(P3)+O(D1) | (9) |
e+O2→e+O(P3)+O(P3) | (10) |
e+O2→e+O(P3)+O(S1) | (11) |
N2(A)+O2→N2(X)+O+O | (12) |
e+O→e+O(3p5P). | (13) |
The first way needs a high excitation threshold energy (about 16 eV) which is much higher than that of the N2(C) product from direct electron collisions with N2 (about 11 eV) [35, 37]. In another way, most of the products stay in the ground state. Therefore, there is a weak emission intensity of oxygen.
The emission intensity is an indicator of the population of excited particles, which can be generated by one or more channels, and this channel depends on the plasma parameters. Figure 6 shows the emission intensities of N2(C-B, 337.1 nm),
As shown in figure 6, the emission intensity of above reactive species is much stronger in smaller electrode gap. The simulation results of electric field intensity as a function of applied voltage at electrode gaps of 15 mm, 20 mm, and 25 mm are shown in figure A1. With the increase of the electrode gap, the electric field intensity decreases significantly. Therefore, at the same applied voltage, the average electric field in a bigger electrode gap is weaker. Thus, there are fewer active particles produced, and the emission intensities of the above active particles significantly decrease with the electrode gap increases from 15 to 25 mm.
The effects of gas flow rate on the emission spectra intensities of N2(C-B),
Rotational temperature and vibration temperature are important parameters of plasma, which can directly affect the chemical reaction rate of the plasma and the surface characteristics of target materials. The vibrational temperature reflects the existence of energy in the excited state of molecular vibration, and the higher vibrational temperature can effectively promote the chemical reaction in plasma. The population of the rotational levels in molecular species is described by rotational temperature. SPECAIR can accurately simulate a variety of molecular transitions including NO, N2,
Figure 9 shows the variations of the vibrational temperature of atmospheric diffuse discharge plasma when the applied voltage increases in different electrode gaps under the gas flow rate of 0.7 l min-1. The discharge gap is bridged completely when the electrode gap is set to 15 and 20 mm, and it is not bridged completely when the electrode gap is set to 25 mm. Therefore, the vibrational temperature has different trends in the three-electrode gap as the applied voltage increases, which is discussed separately in [41]. The vibrational temperature, which indicates electron energy, is reduced when the applied voltage is increased at the electrode gaps of 15 and 20 mm. Due to the complete breakdown of electrode gap, as the applied voltage rises, the current density and conductivity of the discharge area increase, while the discharge current increases [41]. As a result, the reduced electric field that reflects the average electron energy is reduced [28]. On the other hand, as the applied voltage increases, more energy is transferred from the heating electron to the heavy neutral particle, which is part of the reason why the vibration temperature tends to decrease. Similarly, compared with the electrode gap of 20 mm, the discharge current and average electron energy of 15 mm electrode gap are smaller, thus the vibrational temperature is lower [41, 42].
For the electrode gap of 25 mm, with the increase of the applied voltage, the discharge is concentrated in a small area near the needle-ground electrode and the reduced field intensity increases [43, 44]. The electron can obtain higher energy and the discharge zone is small at the electrode of 25 mm. Thus the mean electron energy and the vibrational temperature increase with the increase of voltage, as shown in figure 9.
The rotational temperature as a function of applied voltage at different electrode gaps in the diffuse discharge plasma when the gas flow rate is 0.7 l min-1, is shown in figure 10. With the rise of applied voltage, the rotational temperature obtained by SPECAIR software increases in electrode gaps of 15 and 20 mm. When the applied voltage increasing, the plasma power increases and the rise of energy injected into discharge region makes the collision between electrons and heavy particles more frequent, which makes the rotational temperature rise and the Joule heating effect become more obvious [45]. Thus, the rotational temperature with the electrode gap of 15 mm is higher and the rising trend is more obvious than that in 20 mm.
As can be seen from figure 10, the rotational temperature curves slightly decrease when the applied voltage is increased at the electrode gap of 25 mm. It is caused by the short displacement of gas molecules and ions in the small discharge region which results in a unobvious heating effect. In addition, it can be observed in figures 9 and 10 that the curve error bar with electrode gap of 25 mm is relatively more obvious. This is because the increase of electrode gap reduces the area of the discharge region, which reduces the signal to noise ratio of the emission spectrum.
The effects of gas flow rate on the vibrational temperature and the rotational temperature are shown in figure 11. The applied voltage and electrode gap are kept at 23 kV and 20 mm, respectively. The gas flow rate varies over a relatively small range. Thus, it can be seen that the vibrational temperature and the rotational temperature are almost unaffected by the gas flow rate.
In this paper, an atmospheric pressure large-area diffuse discharge plasma is successfully obtained in an open oxygen-enriched environment by using a specially designed FE and the characteristics of the discharge plasma are studied in diagnosis. The effects of FE on the GST voltage and breakdown voltage are investigated by combining the simulated electrostatic field before breakdown. These findings emphasize that FE can increase the GST voltage and breakdown voltage by altering the electric field distribution, and it can effectively broaden the applied voltage range of stable discharge (corona or diffuse discharge). It is shown that the FE can inhibit GST to a certain extent, which is beneficial to maintain the stability of the discharge. Meanwhile, the emission spectrum of the discharge plasma with the FE is collected, and the vibrational temperature and rotational temperature of N2(C-B) are calculated through it. These data revealed that the emission intensities of N2(C-B),
This work is supported by National Natural Science Foundations of China (Nos. 11965018, 51977023 and 52077026), the Science and Technology Development Fund of Xinjiang Production and Construction of China (No. 2019BC009), the Fundamental Research Funds for the Central Universities of China (No. DUT21LK31), the Key Laboratory Fund of National Defense Science and Technology of China (No. 6142605200303), Science and Technology Plan Project of the Ninth Division of the Crops of China (No. 2021JS003).
[1] |
Semmler M L et al 2020 Cancers 12 269 doi: 10.3390/cancers12020269
|
[2] |
Borges A C et al 2021 Appl. Sci. 11 1975 doi: 10.3390/app11051975
|
[3] |
Qin H B et al 2022 J. Hazard. Mater. 430 128414 doi: 10.1016/j.jhazmat.2022.128414
|
[4] |
Pankaj S K et al 2014 Trends Food Sci. Technol. 35 5 doi: 10.1016/j.tifs.2013.10.009
|
[5] |
Misra N N et al 2011 Food Eng. Rev. 3 159 doi: 10.1007/s12393-011-9041-9
|
[6] |
Misra N N et al 2016 Trends Food Sci. Technol. 55 39 doi: 10.1016/j.tifs.2016.07.001
|
[7] |
Yuan H et al 2018 Surf. Coat. Technol. 344 614 doi: 10.1016/j.surfcoat.2018.03.057
|
[8] |
Desmet T et al 2009 Biomacromolecules 10 2351 doi: 10.1021/bm900186s
|
[9] |
Wang H L et al 2022 Chem. Eng. J. 431 133858 doi: 10.1016/j.cej.2021.133858
|
[10] |
Bogaerts A et al 2020 J. Phys. D: Appl. Phys. 53 443001 doi: 10.1088/1361-6463/ab9048
|
[11] |
Wang R X et al 2022 Plasma Chem. Plasma Process. 42 303 doi: 10.1007/s11090-021-10225-z
|
[12] |
Wang H L et al 2021 J. Phys. D: Appl. Phys. 54 025202 doi: 10.1088/1361-6463/abb8ad
|
[13] |
Park C S, Kim D Y and Kim S O 2014 IEEE Trans. Plasma Sci. 42 2490 doi: 10.1109/TPS.2014.2334552
|
[14] |
Wu S Q et al 2017 Plasma Process. Polym. 14 1700112 doi: 10.1002/ppap.201700112
|
[15] |
Kogelschatz U 2002 IEEE Trans. Plasma Sci. 30 1400 doi: 10.1109/TPS.2002.804201
|
[16] |
Huo W G et al 2014 Phys. Plasmas 21 053505 doi: 10.1063/1.4875723
|
[17] |
Shao T et al 2013 J. Appl. Phys. 113 093301 doi: 10.1063/1.4794031
|
[18] |
Zhang S et al 2019 Spectrochim. Acta A 207 294 doi: 10.1016/j.saa.2018.09.004
|
[19] |
Shi J J and Kong M G 2006 Phys. Rev. Lett. 96 105009 doi: 10.1103/PhysRevLett.96.105009
|
[20] |
Shi J J, Liu D W and Kong M G 2006 Appl. Phys. Lett. 89 081502 doi: 10.1063/1.2338647
|
[21] |
Shi J J, Liu D W and Kong M G 2007 Appl. Phys. Lett. 90 031505 doi: 10.1063/1.2432233
|
[22] |
Kanazawa S et al 2006 Plasma Process. Polym. 3 692 doi: 10.1002/ppap.200600039
|
[23] |
Hu J T et al 2013 Phys. Plasmas 20 083516 doi: 10.1063/1.4817954
|
[24] |
Roman F, Cooray V and Scuka V 1996 J. Electrostat. 37 67 doi: 10.1016/0304-3886(96)00005-8
|
[25] |
Divya Deepak G 2022 Eur. Phys. J. Appl. Phys. 97 39 doi: 10.1051/epjap/2022210275
|
[26] |
Divya Deepak G et al 2017 Rev. Sci. Instrum. 88 013505 doi: 10.1063/1.4974101
|
[27] |
Zhu T H et al 2022 J. Anal. Appl. Pyrolysis 164 105529 doi: 10.1016/j.jaap.2022.105529
|
[28] |
Yang D Z et al 2012 Plasma Sources Sci. Technol. 21 035004 doi: 10.1088/0963-0252/21/3/035004
|
[29] |
Raizer Y P 1991 Gas Discharge Physics (Berlin: Springer)
|
[30] |
Zhang L et al 2019 Nanomaterials 9 1381 doi: 10.3390/nano9101381
|
[31] |
Liu C, Fridman A and Dobrynin D 2019 J. Phys. D: Appl. Phys. 52 105205 doi: 10.1088/1361-6463/aaf8f0
|
[32] |
Yuan H et al 2017 Plasma Sci. Technol. 19 125401 doi: 10.1088/2058-6272/aa8766
|
[33] |
Liu W Z et al 2017 Europhys. Lett. 118 45001 doi: 10.1209/0295-5075/118/45001
|
[34] |
Zhao Z L et al 2016 Spectrochim. Acta A 161 186 doi: 10.1016/j.saa.2016.02.006
|
[35] |
Li Y et al 2020 Plasma Sources Sci. Technol. 29 055004 doi: 10.1088/1361-6595/ab844e
|
[36] |
Herron J T 1999 J. Phys. Chem. Ref. Data 28 1453 doi: 10.1063/1.556043
|
[37] |
Li Y et al 2021 J. Phys. D: Appl. Phys. 54 245206 doi: 10.1088/1361-6463/abed6e
|
[38] |
Zhao Z L et al 2017 Plasma Sci. Technol. 19 064007 doi: 10.1088/2058-6272/aa6679
|
[39] |
Laux C O et al 2003 Plasma Sources Sci. Technol. 12 125 doi: 10.1088/0963-0252/12/2/301
|
[40] |
Luo S Q, Denning C M and Scharer J E 2008 J. Appl. Phys. 104 013301 doi: 10.1063/1.2946718
|
[41] |
Shao T et al 2011 IEEE Trans. Plasma Sci. 39 1881 doi: 10.1109/TPS.2011.2161495
|
[42] |
Zhang L et al 2014 J. Appl. Phys. 116 113301 doi: 10.1063/1.4895982
|
[43] |
Liu F et al 2006 Plasma Chem. Plasma Process. 26 469 doi: 10.1007/s11090-006-9027-1
|
[44] |
Yang D Z et al 2010 J. Phys. D: Appl. Phys. 43 455202 doi: 10.1088/0022-3727/43/45/455202
|
[45] |
Kunhardt E E 2000 IEEE Trans. Plasma Sci. 28 189 doi: 10.1109/27.842901
|
1. | Wang, K., Duan, X., Tian, Y. et al. UiO-66-NH2@tannic acid-assisted solid-phase extraction combined with laser-induced breakdown spectroscopy for sensitive detection of Pb (II) in aquatic products. Spectrochimica Acta - Part B Atomic Spectroscopy, 2025. DOI:10.1016/j.sab.2025.107120 | |
2. | Fu, L., Wang, J., Yao, C. et al. Focused Nanosecond Laser-Induced Bubble Dynamics in Confined Water Within Rigid Spherical Geometry: A Numerical Study | [聚 焦 纳 秒 激 光 在 刚 性 球 内 约 束 水 中 的 击 穿 空 泡 振 荡数 值 研 究]. Zhongguo Jiguang/Chinese Journal of Lasers, 2025, 52(3): 0307208. DOI:10.3788/CJL240901 | |
3. | Cui, S., Liu, Y., Chen, A. et al. Enhancing silicon spectral emission in LIBS using Tesla coil discharge. Plasma Science and Technology, 2024, 26(12): 125504. DOI:10.1088/2058-6272/ad7ddd | |
4. | Cardenas-Escudero, J., Navarro-Villoslada, F., Bellini, G. et al. Determination of bioequivalence between generic and reference drugs using laser-induced breakdown spectroscopy. Analytica Chimica Acta, 2024. DOI:10.1016/j.aca.2024.343253 | |
5. | Zhang, Z., Li, Y., Yang, G. et al. Estimating the grain size of microgranular material using laser-induced breakdown spectroscopy combined with machine learning algorithms. Plasma Science and Technology, 2024, 26(5): 055506. DOI:10.1088/2058-6272/ad1792 | |
6. | Patriarca, M., Barlow, N., Cross, A. et al. Atomic spectrometry update: review of advances in the analysis of clinical and biological materials, foods and beverages. Journal of Analytical Atomic Spectrometry, 2024, 39(3): 624-698. DOI:10.1039/d4ja90010c | |
7. | Cárdenas-Escudero, J., Galán-Madruga, D., Cáceres, J.O. Laser-Induced Breakdown Spectroscopy as an Accurate Forensic Tool for Bone Classification and Individual Reassignment. Applied Spectroscopy, 2024. DOI:10.1177/00037028241277897 |